Spread Spectrum
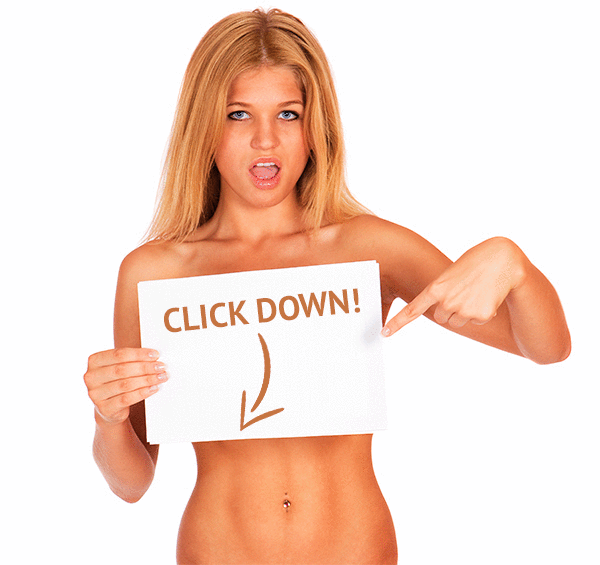
🔞 ALL INFORMATION CLICK HERE 👈🏻👈🏻👈🏻
Spread Spectrum
(3.1) S ( k ) = s 1 c 1 ( k ) + s 2 c 2 ( k ) , k = 1 , … , M ,
(3.2) s ~ 1 = ∑ k = 1 M S ( k ) c 1 * ( k ) = ∑ k = 1 M s 1 c 1 ( k ) + s 2 c 2 ( k ) c 1 * ( k ) = M s 1 + ∑ k = 1 M s 2 c 2 ( k ) c 1 * ( k ) .
(3.3) ∑ k = 1 M s 2 c 1 ( k ) c 2 * ( k ) = 0 .
(3.4) S ( k ) = s 1 c 1 ( k ) v 1 ( k ) + s 2 c 2 ( k ) v 1 ( k ) + s 3 c 1 ( k ) v 2 ( k ) .
(3.5) s ~ 1 = ∑ k = 1 M S ( k ) c 1 * ( k ) v 1 * ( k ) = ∑ k = 1 M s 1 c 1 ( k ) v 1 ( k ) + s 2 c 2 ( k ) v 1 k + s 3 c 1 ( k ) v 2 ( k ) c 1 * ( k ) v 1 * ( k ) = M s 1 + ∑ k = 1 M s 2 c 2 ( k ) c 1 * ( k ) v 1 ( k ) + ∑ k = 1 M s 3 c 1 ( k ) c 1 * ( k ) v 2 ( k ) v 1 * ( k ) = M s 1 + ∑ k = 1 M s 3 v 2 ( k ) v 1 * ( k ) .
URL: https://www.sciencedirect.com/science/article/pii/B978008099969200003X
URL: https://www.sciencedirect.com/science/article/pii/B9780121573454500199
λ 0 = υ / f 0 = 3158 / ( 100 × 10 6 ) = 31.58 × 10 − 6 m = 31.58 micron .
URL: https://www.sciencedirect.com/science/article/pii/B9780121573454500175
URL: https://www.sciencedirect.com/science/article/pii/B9781558608283500060
URL: https://www.sciencedirect.com/science/article/pii/B9780121573454500187
(7.1) r b _ = P d b c _ + j b _ + n b _ .
(7.2) ξ = r b _ c _ T = d b c c _ T + j b _ c _ T + n b _ c _ T = L d b + j b _ c _ T + n b _ c _ T .
URL: https://www.sciencedirect.com/science/article/pii/B9780120471416500070
URL: https://www.sciencedirect.com/science/article/pii/B9781845695347500054
URL: https://www.sciencedirect.com/science/article/pii/B9781785482939500155
URL: https://www.sciencedirect.com/science/article/pii/B9780080507804500138
URL: https://www.sciencedirect.com/science/article/pii/B9780121573454500114
Feature: Applied spread spectrum communication using ultra wide band microwave, and used for very near field.
Thomas Chapman , ... Johan Sköld , in HSPA Evolution , 2015
A spread spectrum communications system is one that is built upon the principle of transmitting information signals over a much wider bandwidth than is strictly necessary for transferring the information. By transmitting over a larger bandwidth, robustness against external narrowband interference is increased, since the wider the bandwidth of any transmitted signal the lower will be the relative influence of interference over a small part of the bandwidth. Although from a single-link point of view, spread spectrum transmission may seem like very inefficient use of spectrum, this is not the case on a system level as spread spectrum techniques allow for simultaneous multiplexing of multiple transmissions in the same bandwidth. Thus, if transmissions to/from K users are multiplexed using K times the bandwidth that would be required without spreading, then the bandwidth utilization is not compromised ( Figure 3.1 ). Furthermore, if the number K of users varies, the spreading applied to each individual user can be varied in such a manner as to ensure that all users are served while bandwidth utilization is maintained. 1
Figure 3.1 . The principle of spread spectrum based user multiplexing.
A variety of spread spectrum techniques exist, such as Frequency Hopping Spread Spectrum (FHSS) and Direct Sequence Spread Spectrum (DSSS). The focus of this chapter is on DSSS.
Consider an information sequence coded/modulated into a sequence of N modulation symbols. In order to spread the sequence using DSSS, an M -chip-long spreading sequence is selected. The M chips of the spreading sequence are replicated N times, with each of the replications being multiplied by one of the N symbols (see Figure 3.2 ). The symbols of this spread sequence are referred to as “chips.” The resulting sequence of N × M chips must be transmitted within the same time as the original N symbol message in order to maintain the data rate. In the frequency domain, this implies an increase in the occupied spectrum for the signal of M times. Hence, M is referred to as the “spreading factor.” In practice, a pulse-shaping filter is then applied, such as a root raised cosine (RRC) filter. The overall occupied bandwidth of the transmitted signal is somewhat larger than M times the bandwidth of the information sequence, as illustrated in Figure 3.3 .
Figure 3.2 . Spreading of an information sequence.
Figure 3.3 . The impact of pulse shape filtering to the occupied bandwidth.
In the absence of other types of channel effects, the receiver can detect the information sequence by means of a synchronized correlation of the spreading sequence with the received sequence ( Figure 3.4 ).
Figure 3.4 . Simple despreading of a spread sequence.
The spreading sequence may in principle be a sequence of random chips. If this is the case, then transmissions to/from different users may be assigned different random sequences. In order to detect a sequence transmitted to or from a particular user, the total received signal, which will consist of aggregation of all users’ sequences, can be correlated with the users’ spreading code at the receiver side. In this case, the target user’s signal will experience a combining gain across the M chips, whereas the other users’ signals will not and hence appear as uncorrelated noise. This combining gain is referred to as spreading gain. This principle is illustrated in Figure 3.5 . In Figure 3.5 a, two users’ signals are spread with different random sequences and combined. In Figure 3.5 b, the combined signal is correlated (that is, multiplied and accumulated) with the same random sequence used for the first user. In this case, the first user’s signal is recovered with a (power) gain factor of M , whereas the multiplication of the second user’s sequence with the first user’s random spreading sequence results in a further random sequence with no gain factor.
Figure 3.5 . Pseudo-noise (PN) code based spreading (a) and despreading (b).
In the following paragraphs, a situation in which two users have information to transmit is considered, in order to simplify the discussion and equations. The discussion may, however, be generalized to consider any number of users.
Spreading of a single modulation symbol is equivalent to
where s 1 is the first user’s modulation symbol, s 2 the second user’s modulation symbol, c i ( k ) the random spreading sequence used for the i th user, and S ( k ) the combined spread sequence. It is assumed that the elements of the spreading sequences are normalized according to | c i ( k )| = 1. The process of recovering the wanted sequence from the spread sequence is known as despreading. Despreading user 1’s signal then becomes
In equation (3.2) , the second term in the right-hand side has a power level M times lower than the first term (note that c x ( k ) ⋅ c x * ( k ) . is always equal to unity).
A problem can arise when the signals from different users are received at very different power levels, for example, because in the uplink one user is close to the base station whereas a second user is not, as shown in Figure 3.6 . In this case, the spreading gain will not be able to suppress the interference from the (strong) second user’s transmission sufficiently and the reception of the first user will experience a poor signal-to-interference ratio because of interference from the second user. This problem is known as the “near-far” problem and is mitigated by the use of power control that keeps the receive power from different transmissions at appropriate levels (depending on the users’ relative data rates).
Figure 3.6 . Near-far problem with DSSS.
There exist better choices of spreading sequence than a random sequence. One example is the Walsh-Hadamard sequences [1] , also known as Orthogonal Variable Spreading Factor (OVSF) sequences. These sequences are available as different lengths and form a hierarchical tree structure, as shown in Figure 3.7 . The sequences have the property that if a sequence from one part of the tree is correlated with another sequence that comes from a different branch of the tree, the resulting cross-correlation is zero
Figure 3.7 . A three-level example of a Walsh code.
The cross code orthogonality also holds true in the general case that OVSF codes of differing lengths (i.e., different M ) from different branches of the OVSF tree (see Figure 3.7 in which C ch,x,y refers to channelization code y with spreading factor x ) are applied; thus, it is possible to apply different spreading factors and transmit data with different symbol rates on different codes. If codes of differing length from the same branch are selected, they will, however, not be orthogonal.
This property of OVSF sequences makes them useful in DSSS systems, because the use of different OVSF sequences for different users allows for different information sequences to be transmitted, at least in principle, without experiencing any cross-user interference when correlation is applied at the receiver. This is different from the case of random codes in which the cross-user interference is only suppressed by the spreading gain. A key requirement, though, for maintaining orthogonality between OVSF codes is that the codes are exactly time synchronous. In some situations, in particular uplink, it may not be feasible to time synchronize transmissions and thus the orthogonality between the codes is not preserved.
The use of orthogonal spreading sequences, such as Walsh-Hadamard, is a powerful technique, because (in the absence of channel effects) users do not interfere with one another. However, the amount of available codes is limited and may not be sufficient to support all users and all cells in a cellular communications system. Typically, spreading will involve a mixture of orthogonal and non-orthogonal codes. Groups of users may share orthogonal codes in a Walsh-Hadamard tree. After spreading, a process called scrambling may be applied in which each group is further multiplied with another random sequence, but not spread. Assuming that all codes at the transmitter are time aligned, then codes that are spread with the same sequence will remain orthogonal, but codes that are spread using different sequences are no longer orthogonal. The scrambling sequences may be random, but more usefully they can be selected to obtain good cross-correlation properties or to facilitate more efficient receiver implementations.
Consider a scenario with three users. In this example, the first two users’ modulation symbols are spread with different orthogonal codes but the same scrambling code v 1 ( k ). The third user’s sequence is spread with the same spreading sequence as user 1 but a different scrambling code v 2 ( k ). It is assumed that the elements of the scrambling sequences are normalized to unity, | v i ( k )| = 1. The combined spread sequence becomes
The symbol s 1 may be recovered by correlation with both the spreading and scrambling code
The first user experiences interference from the third user but not from the second user.
Frequency hopping (FH) spread-spectrum communications techniques are used to provide low error-rate performance in the presence of jamming or high interference levels. In frequency hopping radar systems, synthesizers are required that will provide a large number F of discrete frequencies with good spectral purity in the range 50 ≤ F ≤ 10,000, with circuit bandwidths ranging from 10 MHz to 500 MHz. Fast hopping is of particular interest for countering repeat jamming.
In FH operation, the available bandwidth is divided into a number of contiguous sidebands. Band spreading is then achieved by transmitting successive constant duration pulses on pseudo-randomly (PN) selected subchannel carrier frequencies. The circuit bandwidth should also be programmable to cater for single or multiple carrier frequencies as well as different hopping rates.
While the multimode SAW oscillator may be satisfactory for hopping over a limited number of frequencies, another approach is required for large F and/or very short hopping times. The SAW-based synthesizer employing chirp filter mixing, as now described, represents an efficient and elegant technique for attaining these requirements [ 23 ].
Figure 13.9 shows the structure of a SAW transducer with constant acoustic aperture, for correlation of a five-chip Barker code with (11101) sequence, together with the distribution of surface wave for maximum correlation. Barker sequences form a class of binary codes that have correlation functions with associated peak-to-sidelobe voltage ratios of 20 log 10 N c . True Barker sequences only exist up to length 13, as given in Table 13.1 . The 13-chip Barker code depicted in Fig. 13.6 represents the highest perfect code known, with equal amplitude sidelobes [ 8 ]. Longer sequences may be generated by further encoding each chip by another Barker sequence [ 9 ], [ 10 ]. Thus if a Barker sequence of N c chips is employed and each individual chip is further encoded with another (and faster) Barker sequence of length B c , the processing power gain will be PG = 10 log 10 ( B c N c ), while the peak-to-sidelobe (voltage) ratio will remain at 20 log 10 N c . (Note that the peak-to-sidelobe ratio for maximal sequence PN codes of length N = 2 n − 1, generated by a shift register with n stages and modulo-2 feedback is 20 log 10 √ N [ 4 ] ).
Figure 13.9 . (a) Narrow-band IDT for correlation of a (11101) five-chip Barker sequence. (b) Underlying SAW waveform distribution for maximum correlation. (c) Time-domain response.
TABLE 13.1 . True Barker Code Sequences
Barker phase coding is used in search radars, where increased range resolution and ranging accuracy are required, while using pulse lengths necessary for detection. They are also used in search radars to improve subclutter visibility. In these applications, however, 22.3-dB sidelobe suppression obtained with a normal 13-chip Barker code limits its application to radar environments with low clutter and low target density. Increased peak-to-sidelobe ratios of over 28 dB in the time response of the correlated output have been obtained for SAW implementations of 13-chip Barker coding, however, by weighting the coded IDT finger lengths [ 11 ].
A SAW tapped delay line with biphase encoding may contain more than one waveform cycle per chip. For the Barker sequence example of Fig. 13.9 employing two cycles per chip, the processing gain corresponds to the chip rate N c , while the number of cycles per chip determines the center frequency f 0 of the input and output SAW IDTs. The cycle number will also affect the correlation loss away from transducer center frequency f 0 .
A SAW-based spread-spectrum communications link employs biphase encoding of a digital message signal. The receiver uses a fixed-code SAW filter of the type shown in Fig. 13.7 , with BPSK finger encoding of the narrow-band output IDT. The biphase code length is N c = 127 chips per message bit, at a chip rate R c = 90 M-chip/s. Moreover, the coded IDT is constructed with C = 2 cycles/chip. Determine (a) the number of finger pairs in the coded IDT, (b) the center frequency of this SAW filter and (c) the processing gain.
Solution: (a) The number of coded finger pairs is N p = CN c = 2 × 127 = 254 finger pairs. (b) The center frequency f 0 of the SAW filter is given by f 0 = CR c = 2 × 90 MHz = 180 MHz. (c) Processing gain is PG = 10 log 10 N c = 10 log 10 (127) = 21 dB.
A SAW filter of the type shown in Fig. 13.8 is fabricated on ST-quartz for the correlation of a fixed BPSK code with 127 chips per message bit. The chip rate is R c = 25 MHz, with C = 4 cycles per chip in the output IDT. Determine (a) the center frequency f 0 of the SAW filter, (b) the number of finger pairs in the input IDT, (c) the separation between adjacent tapped sections in the output IDT and (d) the fractional bandwidth of the input IDT.
Solution: The center frequency is f 0 = CR c = 4 × 25 MHz = 100 MHz. (b) The input IDT must accommodate one chip of code at a time, or four acoustic wavelengths. The number of input IDT finger pairs is then N p = 4. (c) At 100 MHz, the acoustic wavelength is
Walter Ciciora , ... Michael Adams , in Modern Cable Television Technology (Second Edition) , 2004
Code division multiple access (CDMA) is a form of spread spectrum communications that is being used for, among other applications, some second-generation cellular phone systems (others use TDMA). The method is also known as S-CDMA, where the S stands for synchronous . S-CDMA is one of the advanced upstream options specified for DOCSIS 2.0.
Figure 4.21 illustrates the principle of CDMA transmission. The basic modulation is often QAM of some level. The data to be modulated on each axis is exclusive ORed with a higher-speed pseudorandom bit sequence (PRBS), which is also known as a spreading code or spreading sequence . The signal is then modulated, normally using m-ary QAM. The effect is to spread out the carrier over a wider bandwidth than necessary for carriage of the information.
Figure 4.21 . Principle of CDMA Transmission.
At the receiver, the demodulated signal is again exclusive ORed with the same PRBS. The result is a collapse of the transmission bandwidth back to that required to convey the information signal without the PRBS. Recovery of the original signal can then proceed. In the process, any narrowband interference that is added to the signal during transmission is spread, most of it out of the receive channel. In addition, if an impairment, such as a suck-out, affects part of the channel, the signal quality is minimally degraded, because most of the energy lies outside of the affected portion of the passband.(For the benefit of data communications engineers, the term suck-out is used in the cable television industry to mean a narrow frequency-selective reduction in the response of a transmission path.)
Figure 4.21 illustrates that many intentional transmissions may occupy the same frequency band simultaneously without interfering with one another, so long as each uses a different spreading sequence. All signals may be received simultaneously, with each having its own unique despreading sequence applied to separate it from the other signals. As each signal is separated with its despreading sequence, power in the other signals is spread out as if it were background noise. All spreading codes used must have the mutual (i.e., shared) property of orthogonality . That is, they must be noninterfering with each other. The practical limitation on the number of signals that can be transmitted in the same bandwidth is the number of orthogonal spreading codes available. In turn, the number of spreading codes depends on the number of bits in the spreading sequence: The longer the sequence, the more orthogonal codes exist. Spreading code design is a complex subject.
The type of spread spectrum system illustrated here is called direct sequence spread spectrum. There exists another practical spread spectrum technique, known as frequency hopping spread spectrum. In this technique, the frequency on which the digital signal is transmitted is moved multiple times during the transmission of one bit. The receiver follows the transmitter's spectrum hopping. One can see intuitively that a narrow band of interference present at one or a few of the frequencies in the hopping sequence will have little effect on the received signal.
With any spread spectrum approach, the total spectrum occupied by the signal is much wider than what would be occupied by the signal without spreading. Spectrum efficiency must be improved by transmitting many signals in the same spectrum at the same time.
This chapter focuses on real-time SAW convolvers for programmable matched filtering, such as in spread-spectrum communications. This chapter examines three types of real-time convolvers. The first
Evil Angel Anal Mike Adriano
Secretary 2022
Camping Outdoor