Light Hole
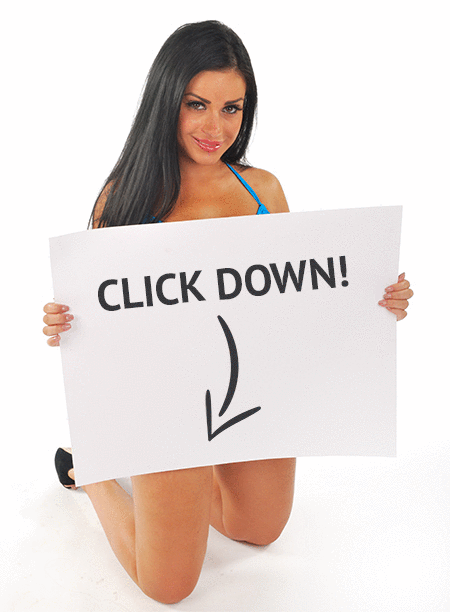
💣 👉🏻👉🏻👉🏻 ALL INFORMATION CLICK HERE 👈🏻👈🏻👈🏻
Thank you for visiting nature.com. You are using a browser version with limited support for CSS. To obtain the best experience, we recommend you use a more up to date browser (or turn off compatibility mode in Internet Explorer). In the meantime, to ensure continued support, we are displaying the site without styles and JavaScript.
A light-hole exciton is a quasiparticle formed from a single electron bound to a single light hole. This type of fundamental excitation, if confined inside a semiconductor quantum dot, could be advantageous in quantum information science and technology. However, it has been difficult to access it so far, because confinement and strain in conventional quantum dots favour a ground-state single-particle hole with a predominantly heavy-hole character. Here we demonstrate the creation of a light-hole exciton ground state by applying elastic stress to an initially unstrained quantum dot. Its signature is clearly distinct from that of the well-known heavy-hole exciton and consists of three orthogonally polarized bright optical transitions and a fine-structure splitting of hundreds of microelectronvolts between in-plane and out-of-plane components. This work paves the way for the exploration of the fundamental properties and of the potential relevance of three-dimensionally confined light-hole states in quantum technologies.
All prices are NET prices.
VAT will be added later in the checkout.
Tax calculation will be finalised during checkout.
Get time limited or full article access on ReadCube.
Zrenner, A. et al. Coherent properties of a two-level system based on a quantum-dot photodiode. Nature 418, 612–614 (2002).
Ramsay, A. J. A review of the coherent optical control of the exciton and spin states of semiconductor quantum dots. Semicond. Sci. Technol. 25, 103001 (2010).
Greilich, A. et al. Mode locking of electron spin coherences in singly charged quantum dots. Science 313, 341–345 (2006).
Press, D., Ladd, T. D., Zhang, B. & Yamamoto, Y. Complete quantum control of a single quantum dot spin using ultrafast optical pulses. Nature 456, 218–221 (2008).
Nowack, K. C., Koppens, F. H. L., Nazarov, Y. V. & Vandersypen, L. M. K. Coherent control of a single electron spin with electric fields. Science 318, 1430–1433 (2007).
Laurent, S. et al. Electrical control of hole spin relaxation in charge tunable InAs/GaAs quantum dots. Phys. Rev. Lett. 94, 147401 (2005).
Gerardot, B. D. et al. Optical pumping of a single hole spin in a quantum dot. Nature 451, 441–444 (2008).
Greve, K. D. et al. Ultrafast coherent control and suppressed nuclear feedback of a single quantum dot hole qubit. Nature Phys. 7, 872–878 (2011).
Michler, P. et al. A quantum dot single-photon turnstile device. Science 290, 2282–2285 (2000).
Akopian, N. et al. Entangled photon pairs from semiconductor quantum dots. Phys. Rev. Lett. 96, 130501 (2006).
Salter, C. L. et al. An entangled-light-emitting diode. Nature 465, 594–597 (2010).
Vrijen, R. & Yablonovitch, E. A spin-coherent semiconductor photo-detector for quantum communication. Physica E 10, 569–575 (2001).
Sleiter, D. & Brinkman, W. F. Using holes in GaAs as qubits: An estimate of the Rabi frequency in the presence of an external rf field. Phys. Rev. B 74, 153312 (2006).
Kosaka, H. et al. Spin state tomography of optically injected electrons in a semiconductor. Nature 457, 702–705 (2009).
Reiter, D. E., Kuhn, T. & Axt, V. M. Coherent control of a single Mn spin in a quantum dot via optical manipulation of the light hole exciton. Phys. Rev. B 83, 155322 (2011).
Schmidt, K. H., Medeiros-Ribeiro, G., Oestreich, M., Petroff, P. M. & Döhler, G. H. Carrier relaxation and electronic structure in InAs self-assembled quantum dots. Phys. Rev. B 54, 11346–11353 (1996).
Karlsson, K. F. et al. Fine structure of exciton complexes in high-symmetry quantum dots: Effects of symmetry breaking and symmetry elevation. Phys. Rev. B 81, 161307(R) (2010).
Besombes, L., Kheng, K. & Martrou, D. Exciton and biexciton fine structure in single elongated islands grown on a vicinal surface. Phys. Rev. Lett. 85, 425–428 (2000).
Belhadj, T. et al. Impact of heavy hole-light hole coupling on optical selection rules in GaAs quantum dots. Appl. Phys. Lett. 97, 051111 (2010).
Li, L. et al. Control of polarization and dipole moment in low-dimensional semiconductor nanostructures. Appl. Phys. Lett. 95, 221116 (2009).
Ridha, P. et al. Polarization properties of columnar quantum dots: Effects of aspect ratio and compositional contrast. IEEE J. Quant. Electron. 46, 197–204 (2010).
Troncale, V., Karlsson, K. F., Pelucchi, E., Rudra, A. & Kapon, E. Control of valence band states in pyramidal quantum dot-in-dot semiconductor heterostructures. Appl. Phys. Lett. 91, 241909 (2007).
Huo, Y. H., Rastelli, A. & Schmidt, O. G. Ultra-small excitonic fine structure splitting in highly symmetric quantum dots on GaAs(001) substrate. Appl. Phys. Lett. 102, 152105 (2013).
Owen, D. L., Lackner, D., Pitts, O. J., Watkins, S. P. & Mooney, P. M. In-place bonding of GaAs/InGaAs/GaAs heterostructures to GaAs(001). Semicond. Sci. Technol. 24, 035011 (2009).
Zander, T. et al. Epitaxial quantum dots in stretchable optical microcavities. Opt. Express 17, 22452–22461 (2009).
Ding, F. et al. Tuning the exciton binding energies in single self-assembled InGaAs/GaAs quantum dots by piezoelectric-induced biaxial stress. Phys. Rev. Lett. 104, 067405 (2010).
Bir, G. L. & Pikus, G. E. Symmetry and Strain-induced Effects in Semiconductors (Wiley, 1974).
Meier, F. & Zakharchenva, B. P. (eds) Optical Orientation (Modern Physics in Condensed Matter Science, Vol 8, Elsevier Science, 1984).
Bayer, M. et al. Fine structure of neutral and charged excitons in self-assembled In(Ga)As/(Al)GaAs quantum dots. Phys. Rev. B 65, 195315 (2002).
Witek, B. J. et al. Measurement of the g-factor tensor in a quantum dot and disentanglement of exciton spins. Phys. Rev. B 84, 195305 (2011).
Bester, G., Nair, S. & Zunger, A. Pseudopotential calculation of the excitonic fine structure of million-atom self-assembled In1−xGaxAs/GaAs quantum dots. Phys. Rev. B 67, 161306(R) (2003).
Bester, G. Electronic excitations in nanostructures: An empirical pseudopotential based approach. J. Phys. Condens. Matter 21, 023202 (2009).
Rastelli, A. et al. Hierarchical self-assembly of GaAs/AlGaAs Quantum dots. Phys. Rev. Lett. 92, 166104 (2004).
Tonin, C. et al. Polarization properties of excitonic qubits in single self-assembled quantum dots. Phys. Rev. B. 85, 155303 (2012).
Trotta, R. et al. Nanomembrane quantum-light-emitting diodes integrated onto piezoelectric actuators. Adv. Mater. 24, 2668–2672 (2012).
We acknowledge P. Atkinson, Ch. Deneke, D. J. Thurmer and R. Engelhard for assistance with the molecular beam epitaxy, D. Grimm, B. Martin and S. Harazim for assistance in clean room maintenance, and G. Katsaros and R. Rezaev for fruitful discussions. This work was financially supported by the BMBF project QuaHL-Rep (Contracts no. 01BQ1032 and 01BQ1034), the DFG FOR730, the FOM (VIDI Grant), the European Union Seventh Framework Programme 209 (FP7/2007-2013) under Grant Agreement No. 601126 210 (HANAS) and the ERANET project QOptInt.
Institute for Integrative Nanosciences, IFW Dresden, Helmholtzstraße 20, 01069 Dresden, Germany
Y. H. Huo, S. Kumar, J. X. Zhang, E. Zallo, R. Trotta, F. Ding, A. Rastelli & O. G. Schmidt
Kavli Institute of Nanoscience, Delft University of Technology, Lorentzweg 1, 2628CJ Delft, The Netherlands
B. J. Witek, N. Akopian & V. Zwiller
Max-Planck-Institute for Solid State Research, Heisenbergstraße 1, 70569 Stuttgart, Germany
J. R. Cardenas, R. Singh & G. Bester
Department of Applied Physics, Eindhoven University of Technology, 5600 MB Eindhoven, The Netherlands
Institute of Semiconductor and Solid State Physics, Johannes Kepler University Linz, Altenbergerstraße 69, 4040 Linz, Austria
R. Grifone, D. Kriegner, R. Trotta, J. Stangl & A. Rastelli
Center for Advancing Electronics Dresden (CfAED), Dresden University of Technology, 01067 Dresden, Germany
You can also search for this author in PubMed Google Scholar
You can also search for this author in PubMed Google Scholar
You can also search for this author in PubMed Google Scholar
You can also search for this author in PubMed Google Scholar
You can also search for this author in PubMed Google Scholar
You can also search for this author in PubMed Google Scholar
You can also search for this author in PubMed Google Scholar
You can also search for this author in PubMed Google Scholar
You can also search for this author in PubMed Google Scholar
You can also search for this author in PubMed Google Scholar
You can also search for this author in PubMed Google Scholar
You can also search for this author in PubMed Google Scholar
You can also search for this author in PubMed Google Scholar
You can also search for this author in PubMed Google Scholar
You can also search for this author in PubMed Google Scholar
You can also search for this author in PubMed Google Scholar
You can also search for this author in PubMed Google Scholar
Y.H.H. grew and processed samples, measured micro-photoluminescence, and analysed data supported by S.K., J.X.Z., E.Z., R.T. and F.D., under supervision of A.R. and O.G.S. B.J.W. carried out micro-photoluminescence in magnetic field supported by N.A. and V.Z. and provided insightful interpretation of the experimental and theoretical results. J.R.C., R.S. and G.B. performed pseudopotential calculations and developed the mesoscopic model. R.G., D.K. and J.S. performed X-ray diffraction measurements. All authors discussed the results and contributed to the manuscript. A.R. conceived and coordinated the project, triggered by V.Z. and N.A.
The authors declare no competing financial interests.
Supplementary Information (PDF 3926 kb)
Huo, Y., Witek, B., Kumar, S. et al. A light-hole exciton in a quantum dot. Nature Phys 10, 46–51 (2014). https://doi.org/10.1038/nphys2799
Advanced Functional Materials (2021)
Nature Physics ISSN 1745-2481 (online)
Sign up for the Nature Briefing newsletter — what matters in science, free to your inbox daily.
I agree my information will be processed in accordance with the Nature and Springer Nature Limited Privacy Policy.
Get the most important science stories of the day, free in your inbox. Sign up for Nature Briefing
We use cookies to make sure that our website works properly, as well as some "optional" cookies to personalise content and advertising, provide social media features and analyse how people use our site. By accepting some or all optional cookies you give consent to the processing of your personal data, including transfer to third parties, some in countries outside of the European Economic Area that do not offer the same data protection standards as the country where you live. You can decide which optional cookies to accept by clicking on "Manage Settings", where you can also find more information about how your personal data is processed.View our privacy policy
Nov 5, 2020 0 I like thisUnlike5
CHASING THE MYSTERIOUS AND ELUSIVE LIGHT HOLE
The semiconductor revolution of the twentieth century gave rise to the microprocessor, spawning a fantastic array of now-familiar electrical devices. As scientists continue to learn more about the physical properties and behavior of semiconductors, we may be on the cusp of a second semiconductor revolution, developing novel materials that will enable a new wave of improved technologies.
Semiconductors are materials with properties intermediate between highly conductive metals, in which free electrons move easily, and non-conducting insulators, in which electrons are tightly bound. The non-conducting electrons in semiconductors occupy a continuum of energy states called the valence band, and they require additional energy to enter the conduction band. Semiconducting materials are classified according to the amount of extra energy a valence electron needs to become conductive in the material. We determine this by observing the energy transitions that occur when light excites the electrons from their ground state to an excited state.
When electrons in a semiconductor acquire enough energy to enter the conduction band, the “missing” electrons in the valence band are referred to as holes. Holes can move through the material in the same way an empty seat in an almost-full row of chairs moves as people shift, one at a time, down the row to fill the empty seat. Similarly, as valence electrons in a semiconductor move to fill a hole, the hole moves in the opposite direction, as shown in Fig 1.
Two of the energy states within the valence band are referred to as the light-hole band and the heavy-hole band; when the electrons occupying these bands become conductive, the remaining holes are light and heavy, respectively. It may seem strange to describe a hole, the absence of an electron, as “heavy” or “light”—by analogy to matter, we can think of the effective mass of a hole as a measure of how much force is required to move it. The light-hole band is notoriously difficult to study, however, because the light-hole and heavy-hole bands are nearly identical in energy. This means that, using traditional optical techniques, the dominant signal of the heavy-hole band tends to obscure the light-hole band (see Fig. 2).
A team at Washington University in St. Louis led by Prof. Sophia Hayes uses an exotic technique known as optically pumped nuclear magnetic resonance (OPNMR), taking advantage of the communication between electrons and nuclei in semiconducting materials to clearly observe transitions from the light-hole band. We begin by using lasers of different colors to excite the electrons in the material at various energies, creating heavy holes in some instances and light holes in others. The conductive electrons have different orientations, depending on whether they originated in the heavy-hole band or the light-hole band, and this affects the nuclei around them. The conduction electrons that originated from the light-hole band cause the nuclear resonance signal from the nuclei to invert, as shown in Fig. 3.
This technique allows for the easy identification and observation of the light-hole energies, and the results we obtain give us new insight into how the band structure of a semiconductor affects its behavior. This, in turn, will enable us to develop semiconductors with desirable properties, which can be used to improve the many electronic devices—like the one you’re using to read this right now—that rely on semiconductor technology.
Fig. 1 (Click to enlarge) A schematic of hole conduction in a semiconductor. Notice that, each time an electron moves to the left to fill the hole, the hole moves to the right. (Photo: Wikimedia Commons)
Fig. 2 (Click to enlarge) The gray lines mark the energies of the light holes in the bulk semiconductor gallium arsenide (GaAs). As you can see, the signal from these electron transitions is faint when we use traditional optical methods.
Fig. 3 (Click to enlarge) The black and red lines show the OPNMR signal intensity obtained using laser light that has been polarized in the clockwise and counterclockwise directions. For both plots, note that the biggest feature we see is at the position of the light hole, where the OPNMR signal reverses direction.
You must be logged in to post a comment.
I agree to my personal data being stored and used as per Privacy Policy
TERMS & CONDITIONS • This site was developed with the support of the National Science Foundation (NSF) under grant DMR 1550737.
Website design and development by Firespring
Porn Hard Minet
Porn Free Horny
Footjob Sexy High Heels
Porn Free Teens Movie
Hole River
A light-hole exciton in a quantum dot | Nature Physics
CHASING THE MYSTERIOUS AND ELUSIVE LIGHT HOLE - Fu…
White hole - Wikipedia
Lightening holes - Wikipedia
Lampade a LED LighHole - LightHole
Hole | SIMON
Blux - Lite Hole C/W - Grupo B.lux
Amazon.com: recessed light hole saw: Tools & Home Improvement
Light Hole
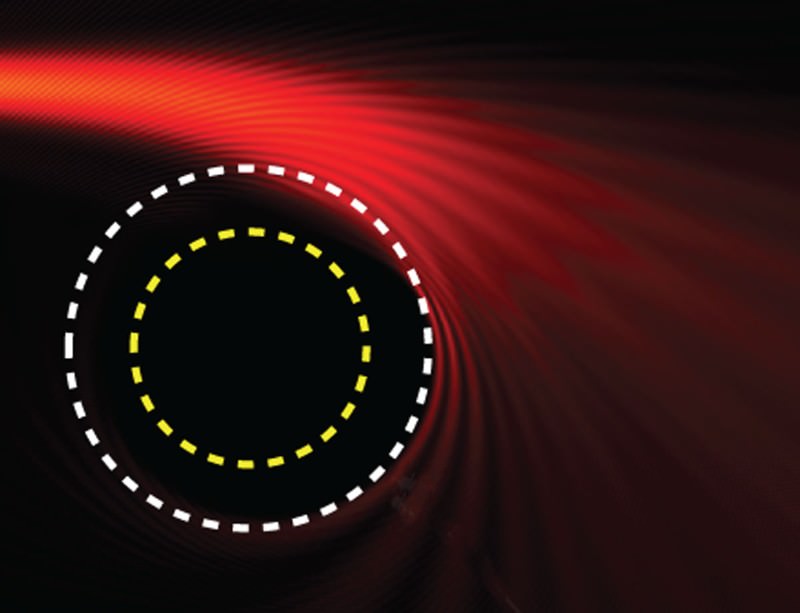
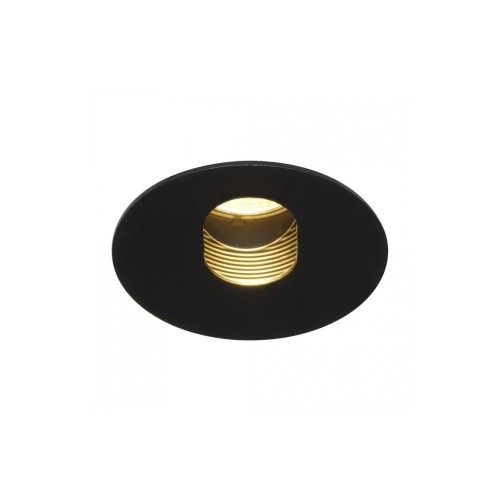
.jpg/1200px-Light_Hole%252C_first_take_(3211900205).jpg)
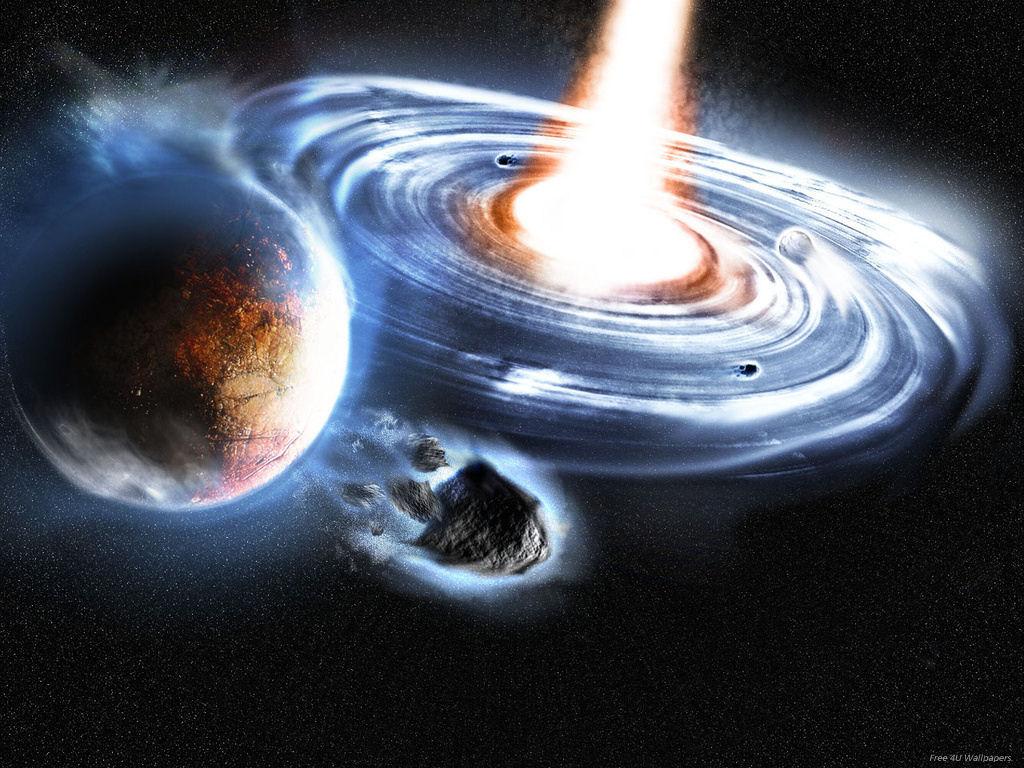



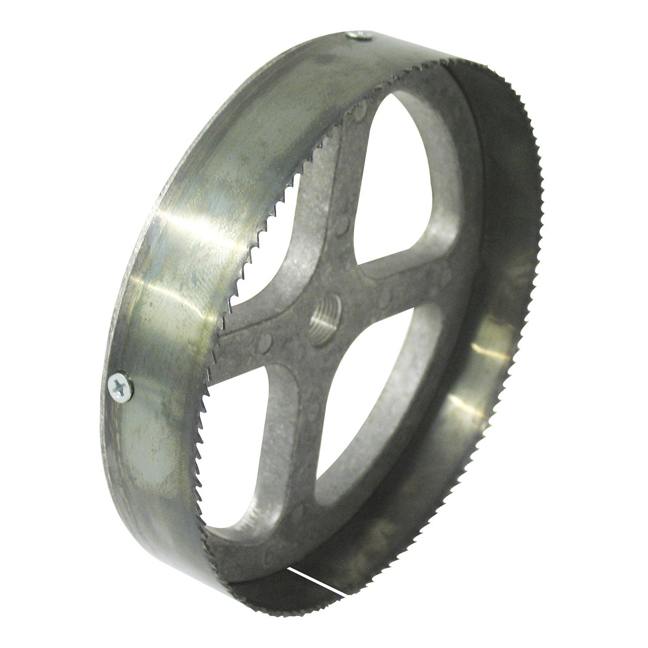
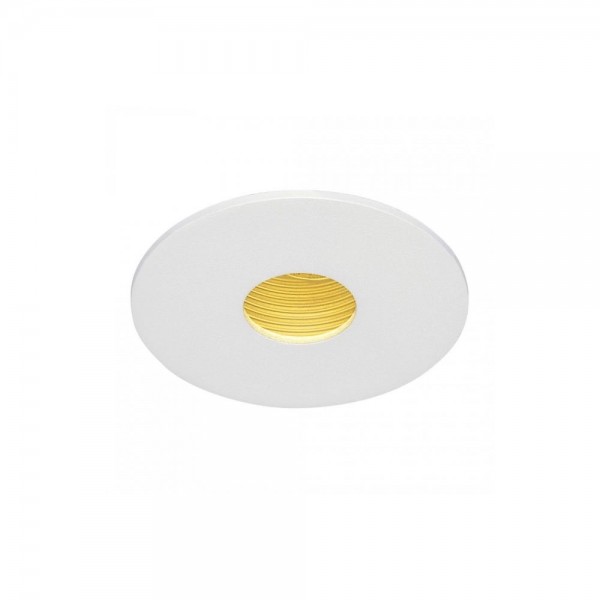


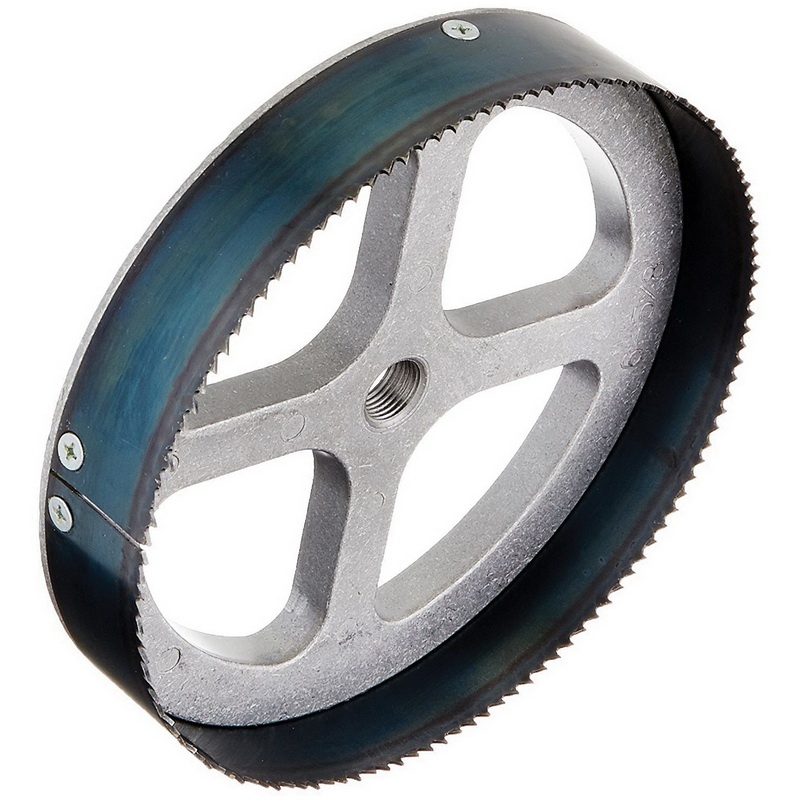

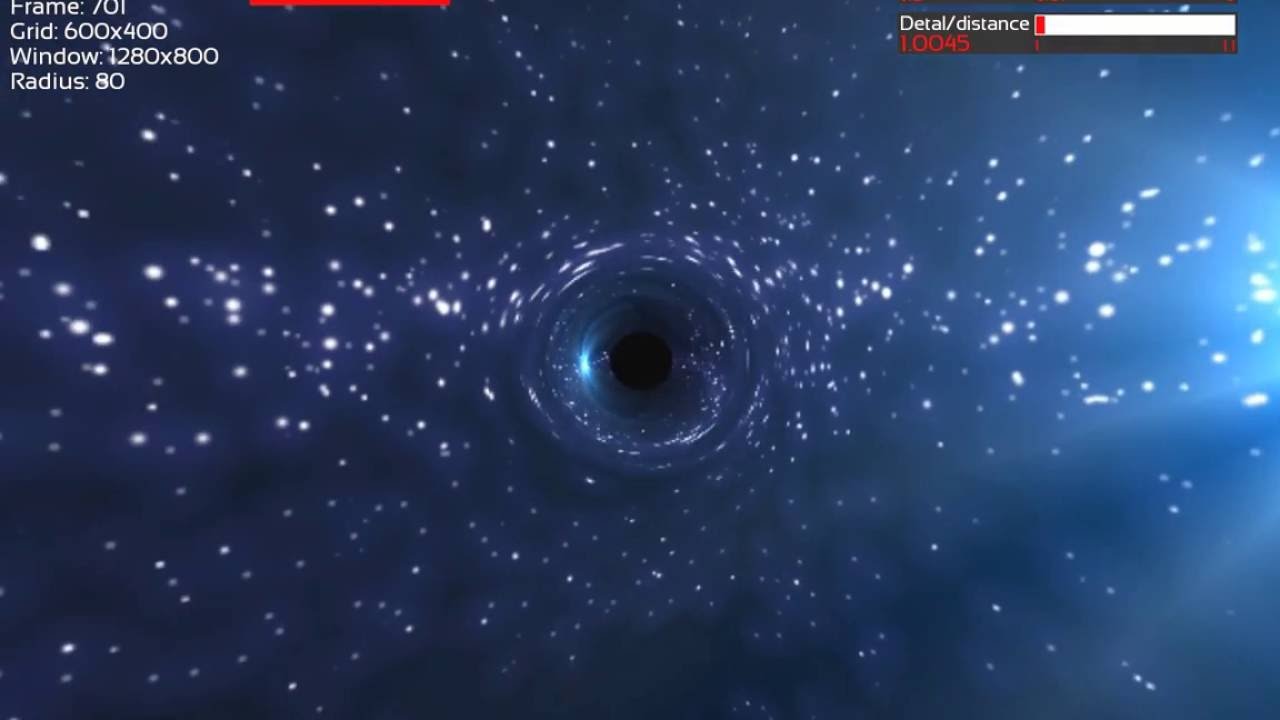






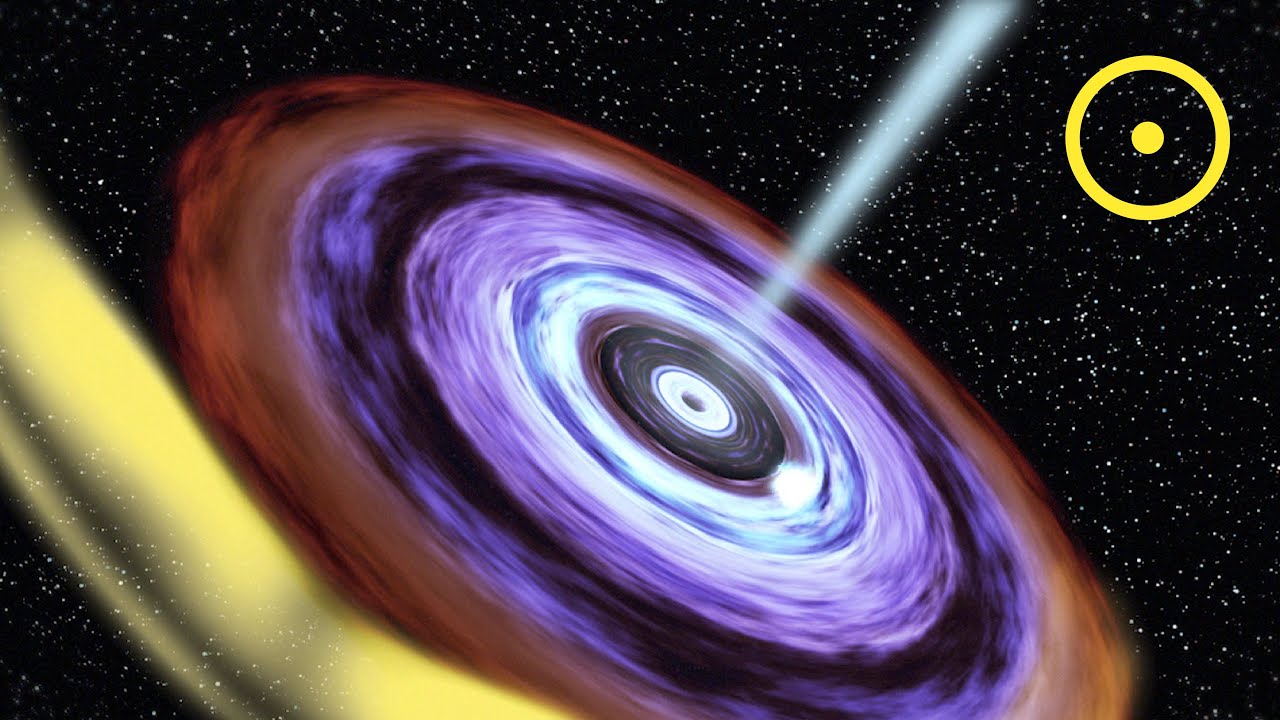


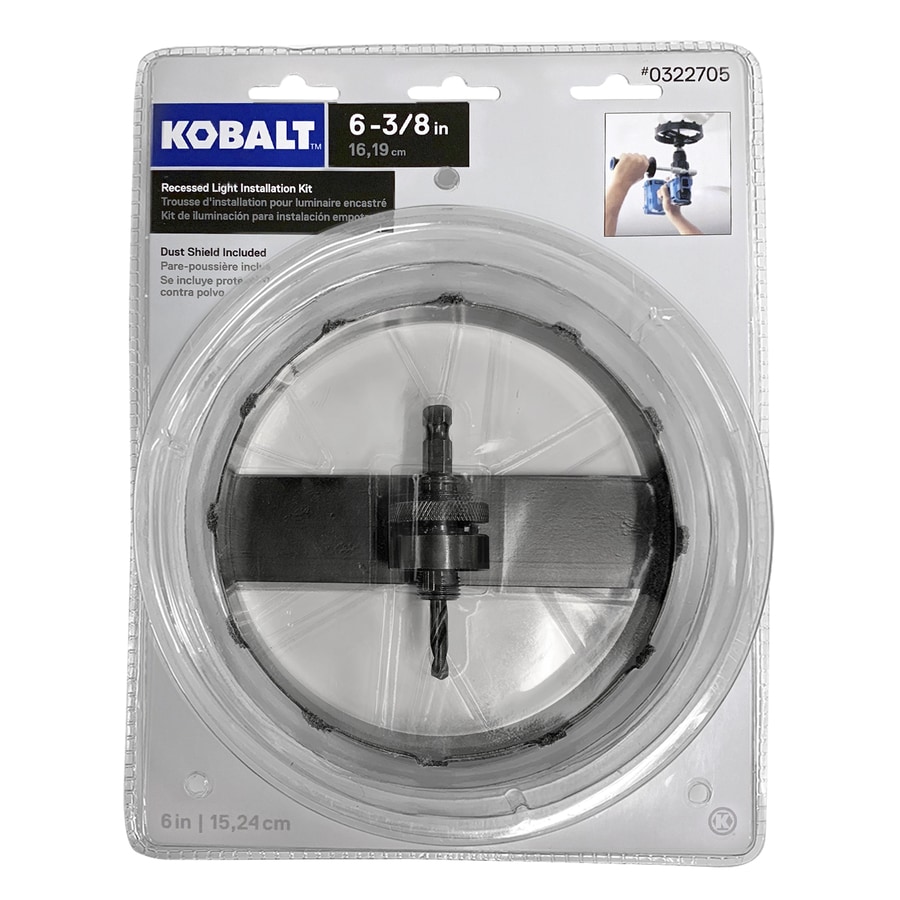
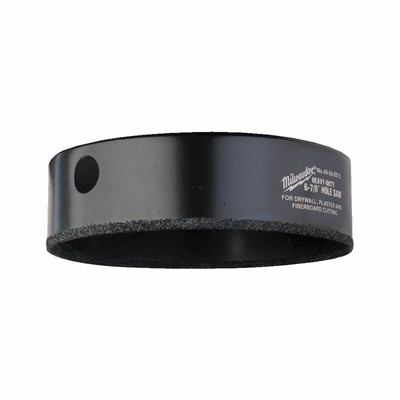

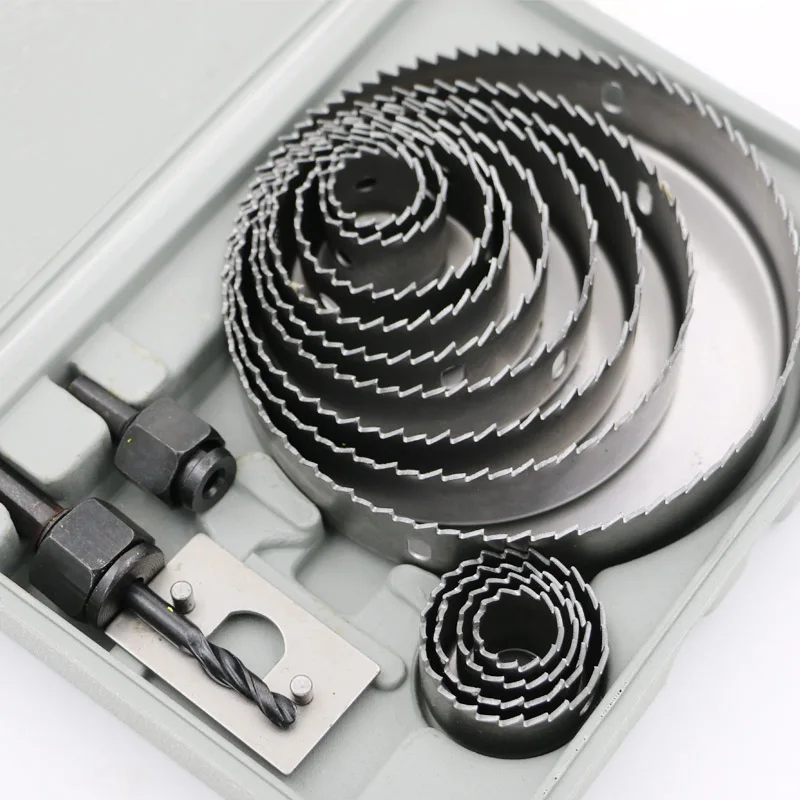

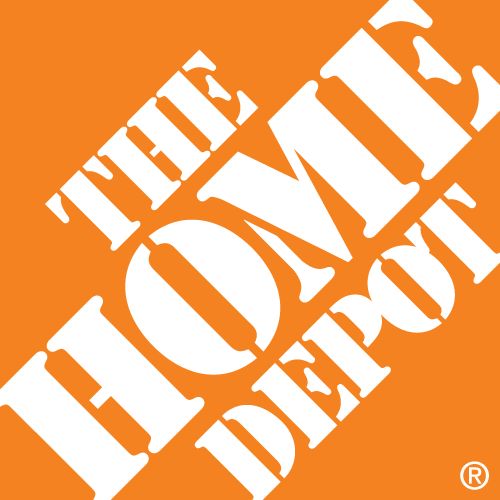
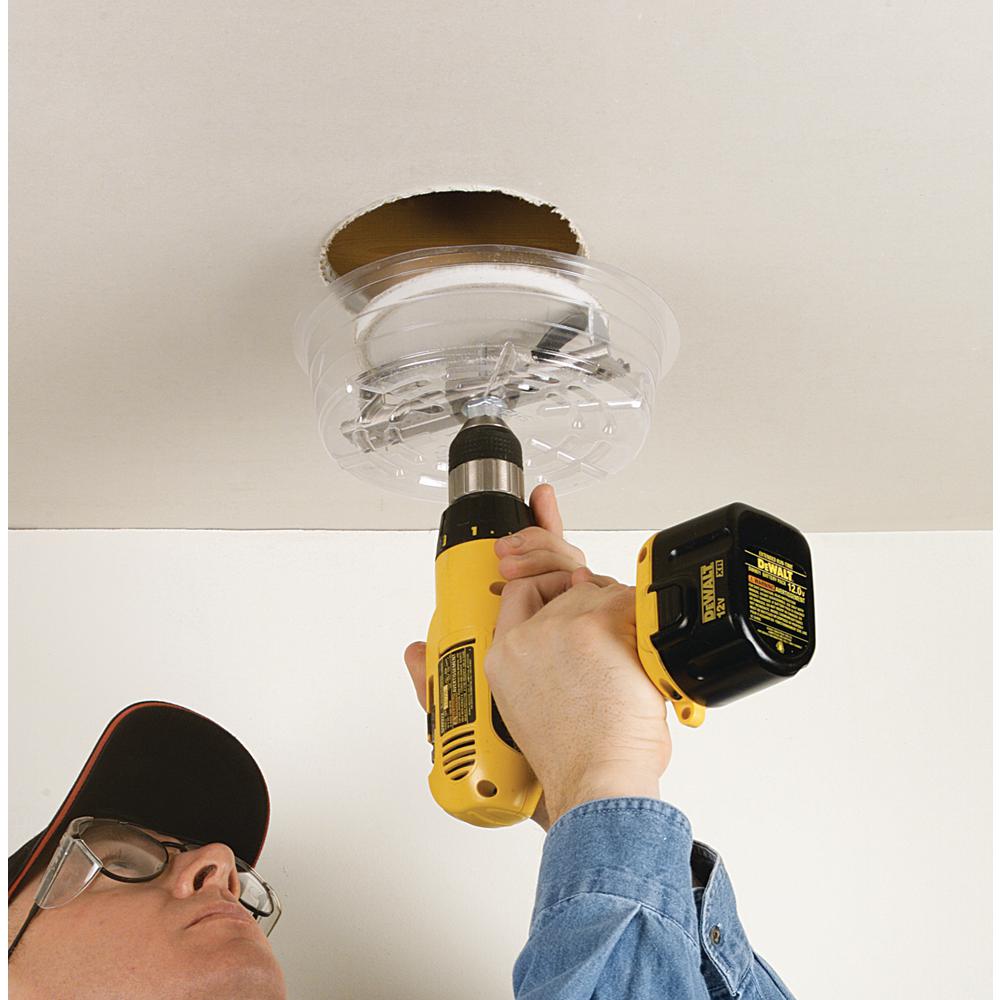
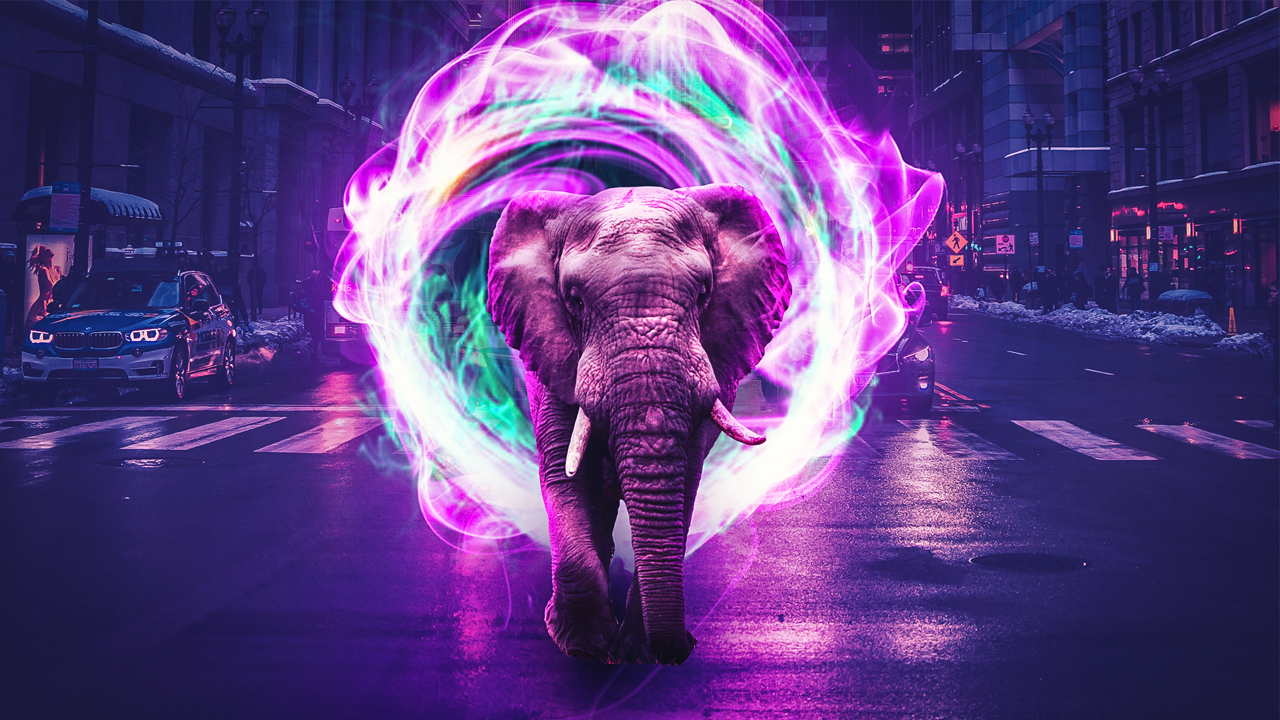


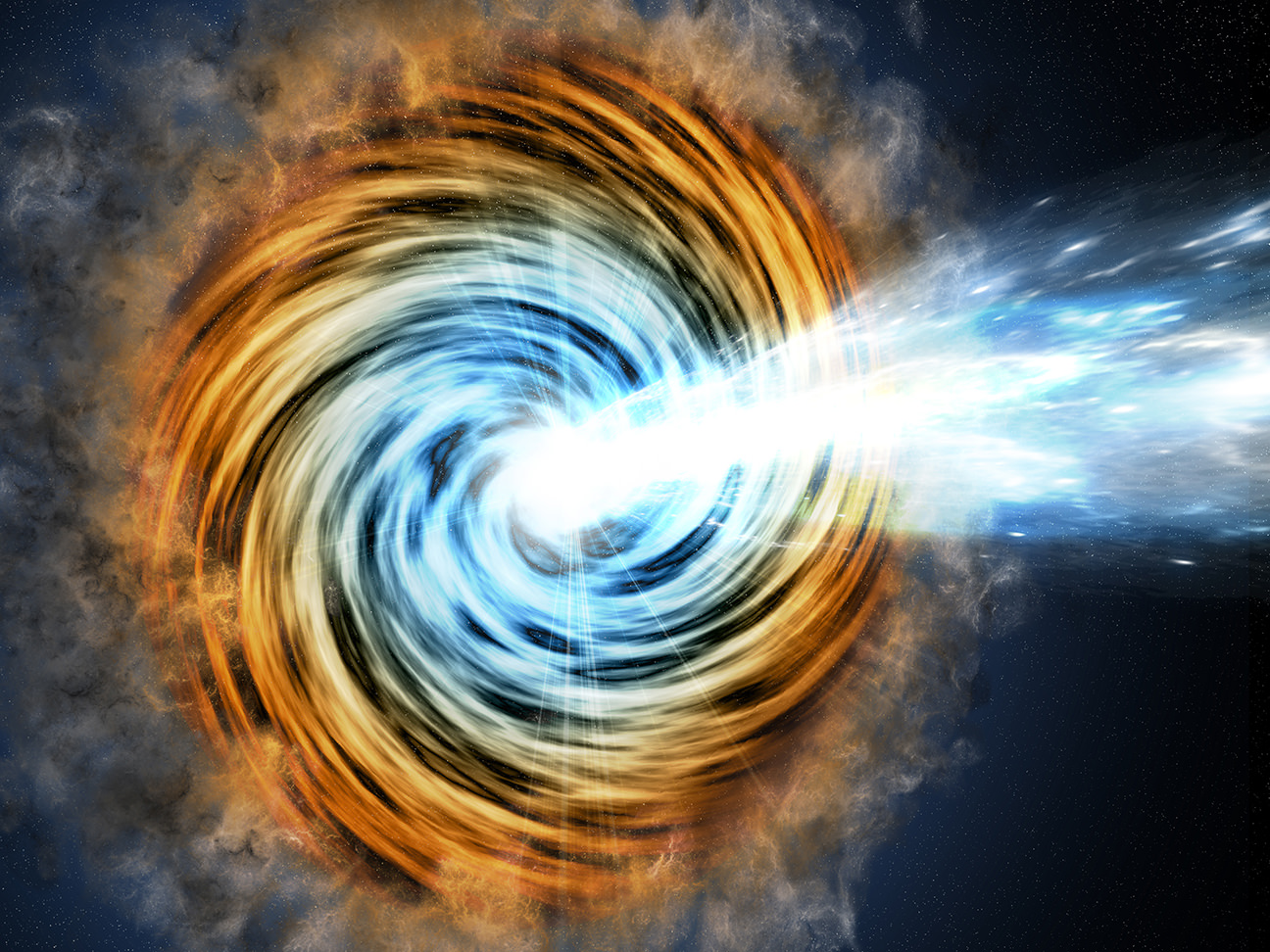



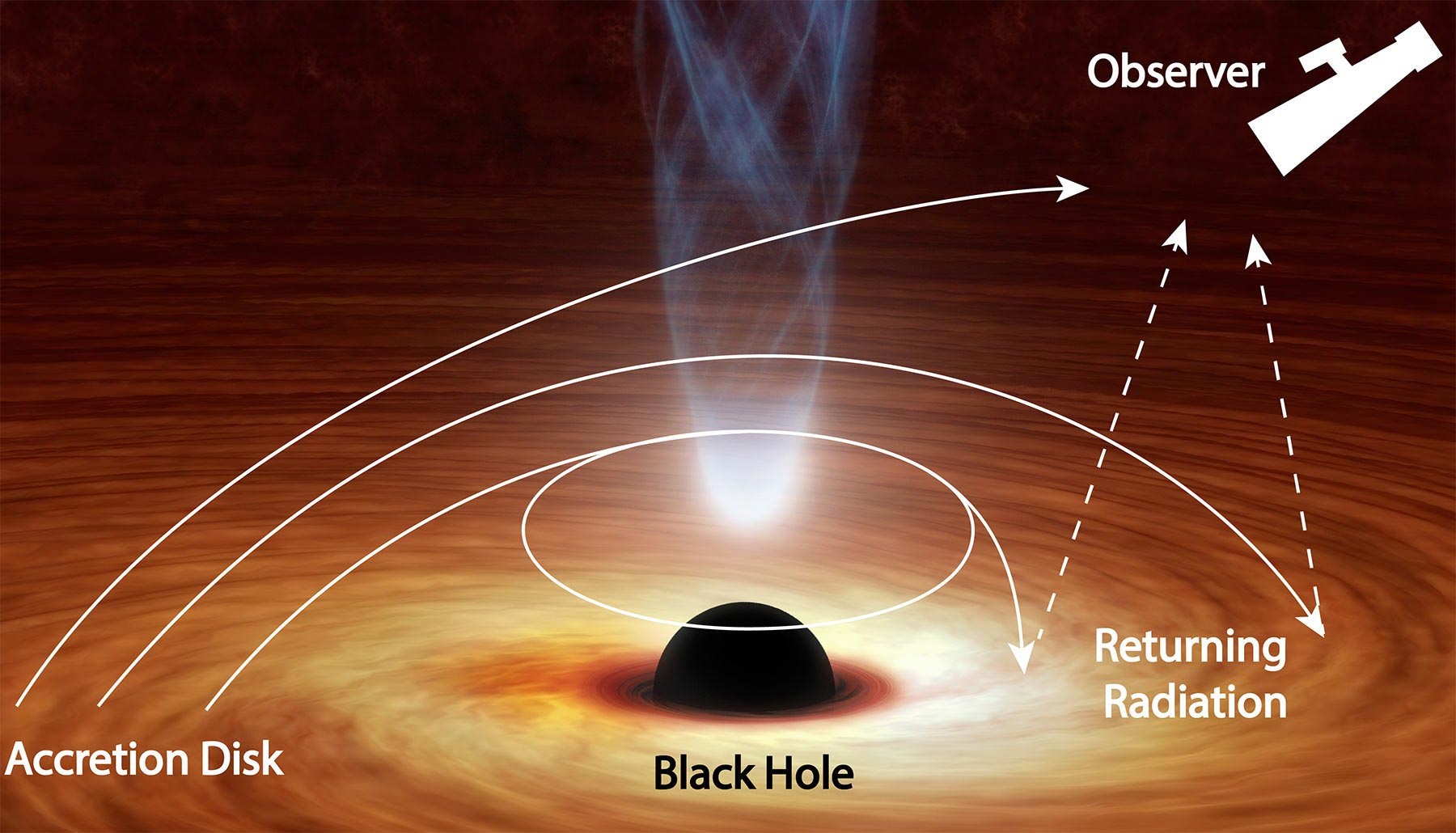
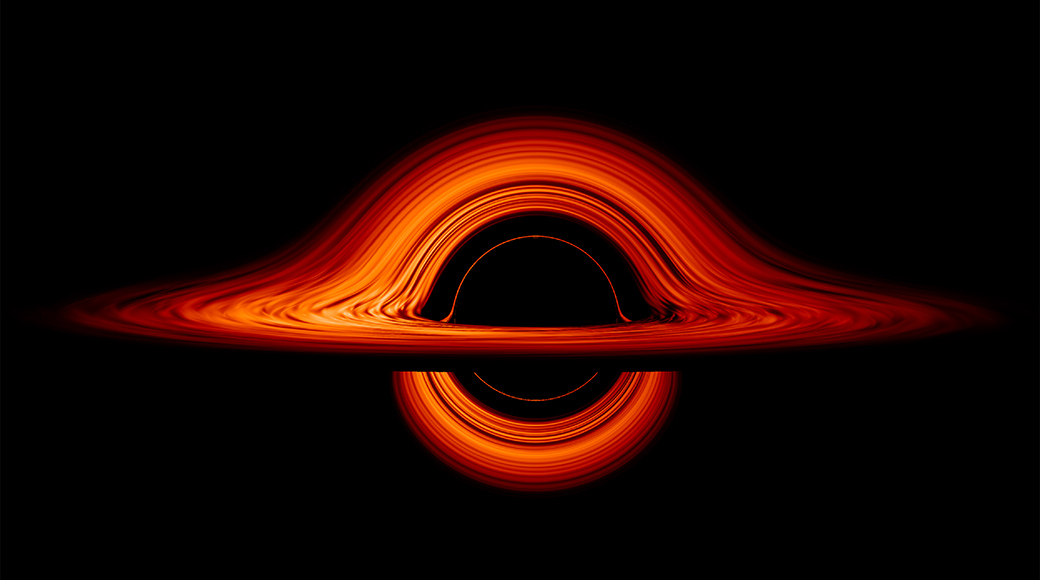
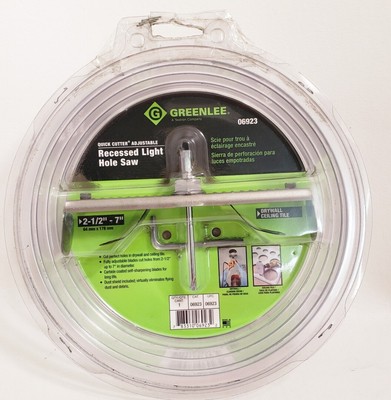



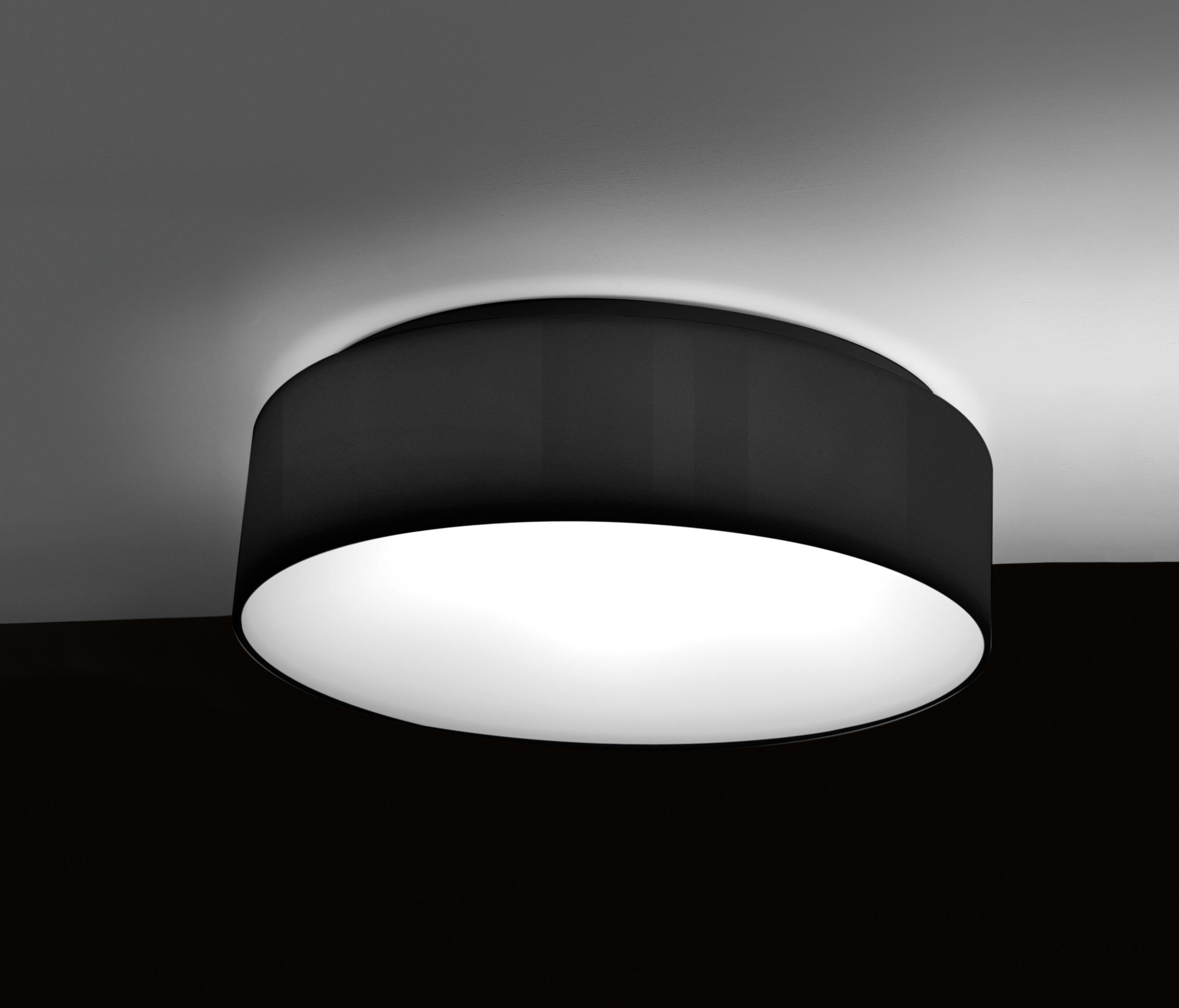



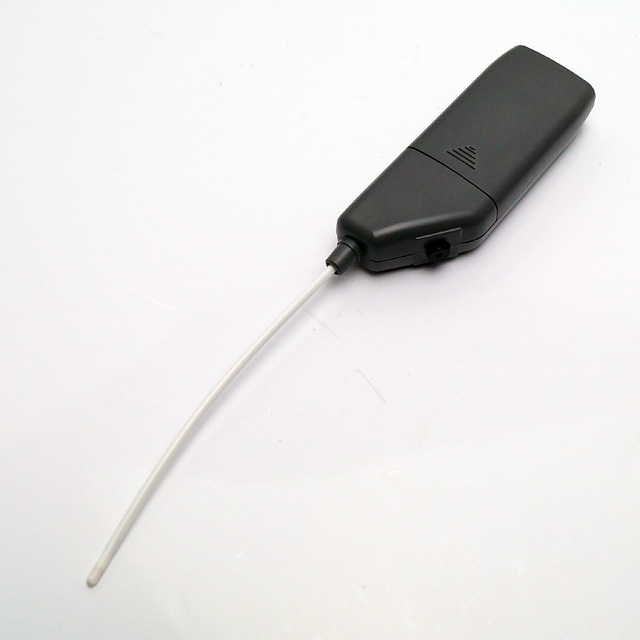









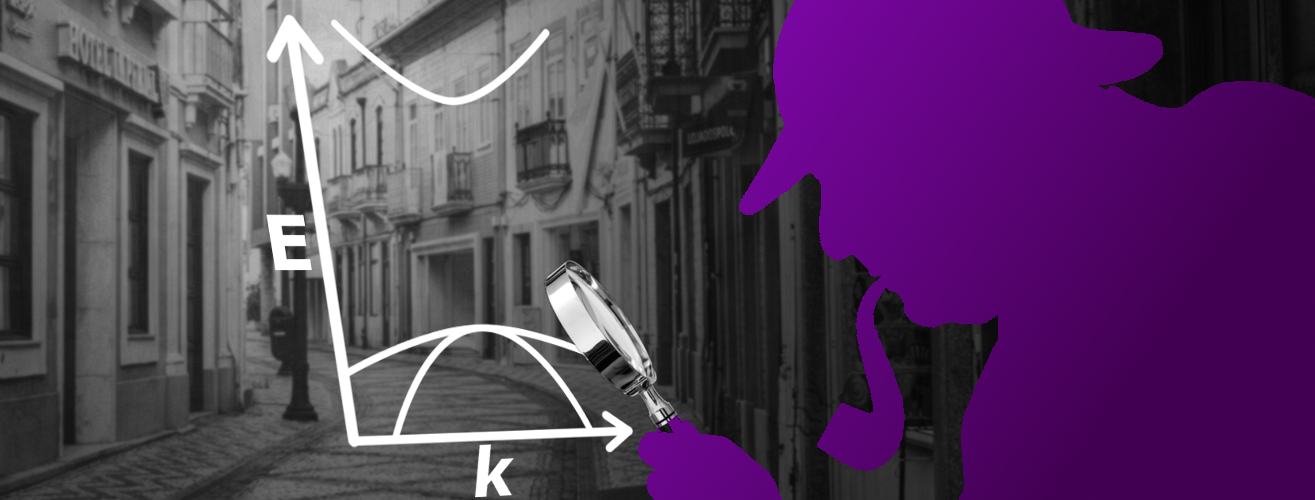
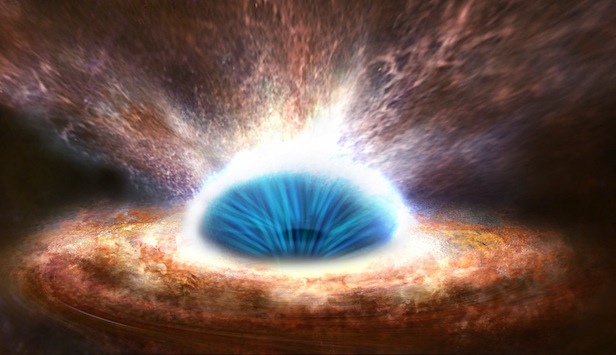