Inductively Coupled Plasma
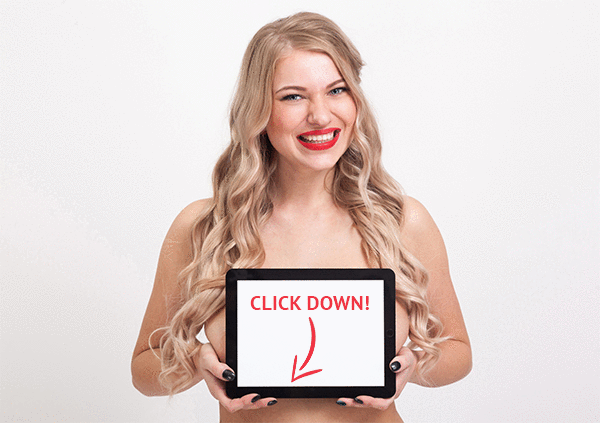
⚡ 👉🏻👉🏻👉🏻 INFORMATION AVAILABLE CLICK HERE 👈🏻👈🏻👈🏻
ICP is an atmospheric pressure ionization method, but unlike the previously mentioned atmospheric pressure ionization methods (ESI, APCI, and APPI), ICP is a hard ionization method, which results in complete sample atomization during sample ionization. An ICP source consists of a sample introduction system (nebulizer and spray chamber that provides the means by which the sample is transmitted into the instrument), an ICP torch, and a radio frequency (RF) coil for the generation of argon plasma, which serves as the ion source, and an interface, which connects the source to the mass spectrometer. In the clinical laboratory, ICP is commonly used for trace element analysis. Among the advantages of ICP are a wide dynamic range and the ability to detect elements at a level of parts per trillion.
URL: https://www.sciencedirect.com/science/article/pii/B9780128154991000107
The ICP–MS is a multielement analysis tool ideally suited for direct coupling with FFF. The ICP torch is capable of vaporizing and ionizing particles in the eluent up to ∼10 μm, and the plasma is then fed into an MS for simultaneous detection of many elements. Quadripole, mass-sector, and time of flight MSs are now available, depending on the sensitivity, mass resolution, and response time required. FFF–ICP–MS yields element-based size distributions. Other element detection systems that have been used include ICP–AESs, electrothermal atomic absorption spectrometers, and very recently laser-induced breakdown spectrometers.
URL: https://www.sciencedirect.com/science/article/pii/B0123693977001515
Inductively coupled plasma (ICP) excitation has revolutionized the field of emission spectroscopy, providing the analyst with a powerful multielement technique with good sensitivity for cadmium at 214.440 or 226.502 nm. Food and food products can be prepared for ICP analysis by either wet oxidation or dry ashing and samples with a wide range of elemental concentrations can usually be handled without the need to dilute or concentrate, since the ICP has a fairly large dynamic range. The current qualities of ICP – high speed, low detection limits (less than 0.01 mg kg−1 for cadmium), and excellent instrument stability – make this technology attractive for a wide range of analytical applications.
ICP-AES is used preferentially due to the possibility of handling a wide range of samples with minimum interference and of quantifying many of the metals, reducing analysis time, and providing a more sensitive determination of refractory elements. The potential of inductively coupled plasma mass spectrometry (ICP-MS) is affected by polyatomic interferences for some mineral elements that can be overcome by different sample introduction methods (hydride generation, flow injection, slurry nebulization) or separating procedures with chelating resins.
URL: https://www.sciencedirect.com/science/article/pii/B012227055X001425
Gregory P. Holmes-Hampton, ... Tracey A. Rouault, in Methods in Enzymology, 2014
ICP-OES, also referred to as ICP-AES (atomic emission spectroscopy), utilizes a plasma torch, a device that causes gas to ionize and become electrically conductive in a state known as plasma. This plasma torch burns at ~ 7000 K, much hotter than the flame in a traditional FAAS setup (~ 2100–2700 K). Passing the argon gas through a magnetic coil and then applying a spark seeds the plasma by atomizing a population of the argon molecules. These charged molecules then have inelastic collisions with the neutral argon that propagates the plasma torch, which depends on the generation of a high-frequency electric current produced by electromagnetic induction known as inductive coupling. The torch will remain lit as long as the magnetic field is present to encourage the movement of the atomized particles of argon. The sample is then introduced as an aerosol via a nebulizer as in AAS. The much higher temperature of the torch in ICP leads to the atomization of essentially all sample molecules, which is not true for AAS.
Once the plasma torch has atomized the sample, the molecules are in an excited state. A detector then measures the light emitted by the sample as it returns to a ground state to determine which elements are present. Compared to AAS, ICP-OES is able to quickly detect many elements (~ 60 per minute, provided you have collected standard curves for these elements), and the instrument's detector determines this rate. ICP-OES also features a much larger dynamic range than AAS, meaning that less sample manipulation is necessary. The biggest discrepancy comparing ICP-OES and AAS is the higher price associated with the purchase of an ICP-OES instrument.
URL: https://www.sciencedirect.com/science/article/pii/B9780128014158000151
Inductively coupled plasma (ICP) sources couple radio frequency energy to an Ar gas stream. The RF energy completely ionizes the argon gas to generate a high-temperature plasma that can effectively ionize elements with very high first ionization potentials. The spread in the kinetic energy of the ions is considerably greater than in the thermal ionization or electron impact ion sources (~ 25 eV vs. ~ 2 eV) and the mass spectrometer with the ICP source requires energy focusing of the ion beam prior to mass separation by the magnetic sector. In addition to the energy filters, isotope ratio mass spectrometers with ICP ion sources have higher mass resolution in order to separate the masses of an element from isobaric interferences. For example, in the measurement of iron isotopes (54Fe, 56Fe, 57Fe, and 58Fe), it is necessary to optically resolve 56Fe+ from the large 40Ar16O+ ion beam. Thus, ICP mass spectrometers are equipped with adjustable entrance slits, which can be changed to achieve the optimum resolution and sensitivity for a particular element. ICP-based mass spectrometers designed for high precision isotope abundance measurements have multiple collectors for simultaneous collection of all isotopic ion currents of interest. As with electron impact and thermal ionization mass spectrometers, the measurement of the relative amounts of isotopes is independent of the fluctuations in the ionization of the sample. Despite the high precision (i.e. repeatability) of the isotope amount ratios measured by the ICP mass spectrometer, the high mass bias (up to several percent per mass unit) means that the ratios are inaccurate. The mass bias arises at the sample and skimmer cones, which form the interface between the plasma torch and the high vacuum of the mass analyzer. Ions of lighter masses are deflected by the high space charge that accumulates in this region. Thus, the ion beam transmitted through the instrument is relatively depleted in the lighter isotopes. The normalization of the measured isotope amount ratios must be done in order to generate meaningful results as the mass bias of the instrument can vary from day-to-day, from element-to-element, and depending on the chemical matrix of the solution being aspirated. Several normalization strategies are employed, including internal normalization using enriched isotopes of the element being measured, the addition of elemental spike of similar mass to the isotopes of interest, or sample-standard bracketing, which is a similar method employed for the calibration of isotope amount ratio measurements made by electron impact ion sources. Unlike the thermal ionization mass spectrometer, the mass bias of the ICP instrument remains relatively stable during the measurement of a sample, which may last for 10–20 minutes. Despite these challenges, the multiple collector inductively coupled plasma isotope ratio mass spectrometer is proving capable of revealing subtle differences in the isotopic abundances of many elements that were previously thought to have uniform terrestrial isotopic composition. This information has resulted in the redefinition of the atomic weights for elements such as Fe and Cu to reflect the variability of the relative amounts of the isotopes in terrestrial reservoirs.
Typically, the liquid analyte is aspirated by a nebulizer and introduced through a spray chamber into the Ar-plasma torch. A measurement session may require 1–3 ml of a solution with an elemental concentration of a few hundred parts per billion.
URL: https://www.sciencedirect.com/science/article/pii/B9780124095472052434
ICP sources have brought about a revolution in multielement analysis. ICPs are generated from radiofrequency (RF) magnetic fields induced by a water- or air-cooled copper coil looped around a quartz tube. The RF magnetic field oscillates at 27.12 or 40.68 MHz, at incident powers ranging from 0.5 to 2.5 kW. Higher powers are usually applied when organic solvents are aspirated. Argon gas flows through a torch, which consists of three concentric tubes usually constructed from fused silica. The plasma is initiated by seeding the argon stream with electrons provided from a Tesla coil. The electrons, detached from the argon atoms, collide with further argon atoms and populate the coil region with positive and negative charges. Because of the magnetic field, the particles flow in a closed annular path. Due to the conductance of the gases in the coil region, the charged particles are heated by inductive coupling to a temperature equaling the ionization temperature of the support gases ∼−7000–8000 K in the case of argon. A chain reaction of collisional ionization occurs, resulting in the formation of the ICP. In practice, the plasma impedance is monitored along with the tube grid current, grid voltage, plate current, and voltage. These data are fed back to a loop to control the plasma power. The configuration of an ICP-AES system is shown in Figure 4.
Figure 4. Schematic of an inductively coupled plasma source for atomic emission spectrometry.
URL: https://www.sciencedirect.com/science/article/pii/B0123693977000297
Inductively coupled plasmas either combined with atomic emission spectrometers (ICP–AES) or mass spectrometers (ICP–MS) where samples are excited using a high-temperature gaseous plasma can be used for elemental analysis. Since the development of ICPs, most applications have required digestion of solid samples with heat and/or strong acids. The coupling of laser-ablation (LA) with ICP–MS has resulted in the development of extremely sensitive microprobes capable of determining most elements of the periodic table. Another advantage of the technique is that LA–ICP–MS is point specific, that is, only the area ablated is subject to analysis. As a result, LA–ICP–MS has become one of the most exciting new fields of research in materials science in general.
URL: https://www.sciencedirect.com/science/article/pii/B0123693977002016
Inductively coupled plasmas were developed in the early 1960s [2]. They are flame-like discharges that can reach up to 10,000 K in temperature and are formed in a stream of argon, though other gases have been used, flowing through an electromagnetic field.
Typically in an ICP the gas flows into three concentric tubes. These tubes are assembled together in what is commonly referred to as the plasma torch (Fig. 12.1). The flowing gas in the outer tube, which is typically in the range of 13–17 L/min, is often referred to as the cool gas and its primary role is to ensure that the high temperature of the plasma does not melt the torch. In addition to this primary function, the cool gas also plays a role in giving the plasma its distinct shape. The flowing gas in the central tube, which is usually in the range of 1 L/min, is commonly referred to as the plasma gas and is actually the gas that gets ionised to form the plasma. Finally, the gas flowing in the innermost ring is called the carrier gas and is used to punch the plasma whilst carrying an aerosol or particulate form of the sample to be analysed.
Fig. 12.1. A schematic of the drawing of an ICP torch.
As shown in Fig. 12.1, one end of the torch is encircled by a coil. A radio-frequency current flows through the coil to create an electromagnetic field in which the argon flows. To light the plasma a high voltage discharge (spark) is passed through the argon. When passing through the electromagnetic field, electrons from the discharge gain energy. Some of these electrons transfer this energy through collisions to the argon atoms in the gas thereby ionising them. This will create sets of ion-electron pairs that are in turn energised in the presence of the electromagnetic field and are made to participate in this cascading method of energy transfer from the coil to the gas. The outcome of this cascade is the formation of a steady-state plasma that is maintained as long as the radio-frequency current is upheld at a sufficient intensity and the gas flow is preserved. The fireball-like argon plasma is characterised by a bluish white emission which is a combination of emissions from the line spectrum of atomic argon and the continuous spectrum of ion-electron recombinations taking place within the plasma.
When formed, the plasma has the shape of a prolate spheroid, and the rapid expansion and acceleration of the argon gas inside it makes it difficult for sample introduction. However, by punching a central channel inside the plasma, its shape is changed into an annular “doughnut” form with the outer “plasma” gas virtually shielding the central “carrier” gas and with very little mixing taking place between the two. As a result, samples can be introduced in a gaseous or aerosol form along this central channel without significantly disturbing the plasma or changing its composition. However, during its stay in this channel, the sample will be efficiently desolvated, atomised, excited and ionised. Hence any chemical species injected into the plasma will be broken down into its constituting elements which are then ionised independently of their original form, but depending mainly on their ionisation energies as predicted by the Saha Equation (see Appendix 1). In fact, most elements in the periodic table will be efficiently converted into singly charged ions. As shown in Fig. 12.2 the efficiency of ionisation, decays as the ionisation energy of the element approaches that of argon (15.8 eV). Hence, elements such as F, Ne and He are so poorly ionised that the technique cannot be used for direct trace level determinations of these elements.
Fig. 12.2. A representation of the efficiency of ionisation of elements in an ICP as a function of their ionisation energy.
URL: https://www.sciencedirect.com/science/article/pii/S156771920380013X
The ICP is generated by coupling the energy from a RF generator into a suitable gas via a magnetic field that is induced through a two or three turn, water-cooled copper coil. The RF energy is normally supplied at a frequency of 27.12 MHz, delivering forward power at between 500 and 2000 W. Two gas flows, usually argon, flow in a tangential manner through the outer tubes of a concentric, three-tube quartz torch that is placed axially in the copper coil. The outer and intermediate gases flow tangentially (i.e., they swirl around as they pass through the torch), so the plasma is continually revolving and has a ‘weak spot’ at the center of its base, through which the inner gas flow, containing the sample, can be introduced. A spark is used to seed the gas with electrons, which then accelerate in the magnetic field and reach energies sufficient to ionize gaseous atoms in the field. Subsequent collisions with other gaseous atoms cause further ionization, and so on to form a self-sustaining plasma. This occurs almost instantaneously. The magnetic field causes the ions and electrons to flow in the horizontal plane of the coil, thereby heating neutral argon by collisional energy exchange, and a hot fireball is produced. The hottest part of the ICP has a temperature between 8000 and 10000 K, which is the temperature of the surface of the Sun, though the analytically useful region is in the tail-flame with a temperature between 5000 and 6000 K.
In the absence of analyte atoms, water, and sample matrix components, the predominant species in an argon ICP will be Ar, Ar+, and e−, though other species can be important when considering analyte ionization mechanisms. The RF energy used to sustain the plasma is only coupled into the outer region of the plasma, so these species are primarily formed in this region and are thermally transferred to the center and circulate between the outer and central regions. Additionally, when the sample is introduced through the axial channel, the center will contain a flow of cooler gas containing the analyte and species derived from the sample matrix and water.
URL: https://www.sciencedirect.com/science/article/pii/B0123693977000364
Although an ICP exhibits a high kinetic temperature, the residence time during which the sample remains in the plasma is limited to a few milliseconds. Consequently, it is necessary to introduce the sample in the form of small liquid or solid particles of the order of a few micrometers to ensure complete volatilization and atomization. This is a serious constraint in the design of the sample introduction system.
In general, solids are introduced in the form of fine particles, either in suspension in a liquid (slurries) or in a gas stream. Spark ablation and laser ablation can also be used for this purpose. Direct introduction of solids, placed in a refractory vessel at the base of the plasma, has also been described.
The most common way for a sample to be introduced into an ICP is a solution. In order to produce fine droplets from a liquid, a nebulizing system is required. To date, the most widely used type is the pneumatic nebulizer, which has three variants: the concentric, the cross-flow, and the V-type. V-nebulizers are less subject to blocking. In using a nebulizer, the aim is to achieve efficient nebulization at a low carrier gas flow rate (<1 l min−1). However, the nebulizers in service today provide droplet distributions centered on ∼20 μm. It is therefore necessary to add a chamber, called a spray chamber, in order to filter out droplets larger than a few micrometers in diameter. The overall efficiency of the nebulizer associated with the spray chamber is very low – only ∼2–5% for liquid consumption in the range 1–2 ml min−1. Currently, micronebulizers, i.e., micronebulizers efficient below 0.2 ml min−1 are commercially available, with efficiency higher than 50%. A pneumatic nebulizer is usually fed via a peristaltic pump to ensure a constant uptake rate irrespective of the viscosity of the liquid.
Ultrasonic nebulizers are free of these difficulties as aerosol production is independent of gas flow, and droplet size is related to ultrasonic frequency. Droplets with diameters of ∼1 μm can be obtained with frequencies above 1 MHz. The efficiency is high enough to require the addition of a desolvation system to eliminate water. The desolvation system consists of an oven associated w
Blacked Anal Hd
Russian Anal Bdsm
Gives Pussy
Michaels Xxx
Https Bikini
Inductively coupled plasma - Wikipedia
Inductively Coupled Plasma - an overview | ScienceDirect ...
Inductively Coupled Plasma - an overview | ScienceDirect ...
Inductively Coupled Plasma Mass Spectrometry (ICP-MS ...
Inductively Coupled Plasma (ICP) - Chemistry LibreTexts
Inductively coupled plasma mass spectrometry - Wikipedia
Inductively Coupled Plasma















.jpg)
































.jpg%3ftype%3dw2)






