Cross Coupled
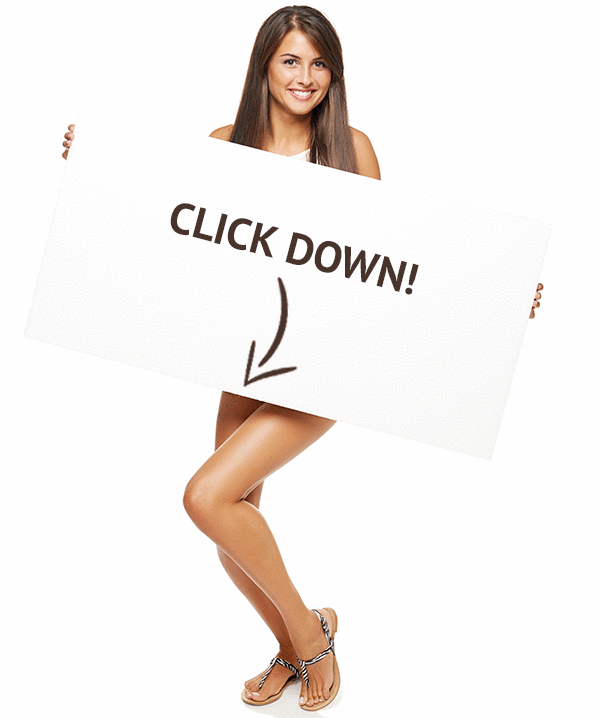
🔞 ALL INFORMATION CLICK HERE 👈🏻👈🏻👈🏻
Cross Coupled
URL: https://www.sciencedirect.com/science/article/pii/B0080437486090897
URL: https://www.sciencedirect.com/science/article/pii/B0080437486090903
URL: https://www.sciencedirect.com/science/article/pii/B0122274105000855
URL: https://www.sciencedirect.com/science/article/pii/B9780444533494001382
URL: https://www.sciencedirect.com/science/article/pii/B9780128092705000212
URL: https://www.sciencedirect.com/science/article/pii/B0080437486011038
URL: https://www.sciencedirect.com/science/article/pii/B9780444538024002086
URL: https://www.sciencedirect.com/science/article/pii/B9780080967011002433
URL: https://www.sciencedirect.com/science/article/pii/B9780128228944000101
URL: https://www.sciencedirect.com/science/article/pii/S0950140110040188
The cross coupling and platform tilt errors result from unintentional motion of the vessel.
I.P. Beletskaya , A.V. Cheprakov , in Comprehensive Coordination Chemistry II , 2003
Cross-coupling of terminal acetylenes used as nucleophiles with aryl or alkenyl halides (referred to as the Sonogashira–Hagihara, or SH, reaction) is a versatile method of synthesis for acetylenic compounds, which are rapidly gaining importance as advanced new materials and building blocks for implementing unusual molecular architectures.
In the SH reaction acetylenes are used as they are, without the prior transformation into organometallic compounds which is required in other methods of sp (M)– sp x coupling. The SH reaction commonly uses a standard protocol with Pd(PPh 3 ) 4 or similar Pd complexes used as precatalysts, a tertiary amine as base, and copper(I) salts as cocatalysts, in a number of media including ethers (THF, dioxane) and dipolar aprotic solvents. The combination of amine and cuprous salt is believed to effect the in situ transformation of the alkyne into a copper(I) acetylide, which is likely to serve as a coupling partner. 142 This interpretation is not flawless, as it is well known that cross-coupling of aryl iodides with terminal acetylenes can be performed in the absence of cuprous cocatalyst, and other bases can be used in place of amines.
The scope of the SH reaction encompasses sp–sp 2 (alkenyl, aryl ( 29 ), 143 heteroaryl) and sp–sp couplings (the modified Cadiot–Chodkiewicz reaction). 142 Iodides are most frequently used as electrophilic coupling partners, though the use of bromides, triflates ( 30 ), 144 or even some reactive chlorides ( 31 ) 145 is also possible. Due to the low steric bulk of the acetylenic unit, as well as its exceptional ability in the transduction of electronic effects, the SH reaction is well suited for construction of new (e.g., star-like) molecular architectures through polysubstitution ( 32 ). 146
In spite of the common conception that Ni catalysts are useless in the Sonogashira reaction, NiCl 2 (PPh 3 ) has been disclosed as being able to catalyze the cross-coupling of aryl iodides with terminal acetylenes in aqueous dioxane, in the presence of CuI. 147
The exclusion of copper from the Sonogashira reaction protocols is a desirable modification for several reasons. The essential simplification of the procedure is very important for large-scale applications. Copper salts also have a well-known ability to promote undesired homocoupling of acetylenes, giving rise to diacetylene by-products, 148 or intramolecular carbopalladation in the case of tethered acetylenes. 149 However, no general copper-free protocol has been developed, though occasionally the cross-coupling with terminal acetylenes is shown to proceed flawlessly in the absence of Cu I salts. It has been reported that Pd(PPh 3 ) 4 as a precatalyst does not require CuI, while PdCl 2 (PPh 3 ) 2 does. 150 Indeed, in copper-free procedures Pd(PPh 3 ) 4 is usually used in the presence of an amine base and an aryl iodide as electrophile, as in the synthesis of rod-like carbohydrate clusters ( 33 ); 151 aryl bromides or triflates can also be used as the electrophile, 152 albeit not as smoothly ( 34 ). 153 Symmetrical tolanes can be prepared in a Cu-free procedure using trimethylsilylacetylene as an acetylene synthon ( 35 ). 154 Silver oxide in conjunction with Bu 4 NF serves in a copper- and amine-free procedure. 155 The other notable Cu-free procedure requires the use of a phase-transfer agent in aqueous MeCN solvent. 156
Apart from Cu I , Zn II salts or Zn metal were also shown to be effective, thus bringing forward an alternative efficient protocol ( 36 ) which may be useful for the applications in which the contamination of products by Cu cannot be tolerated. Such protocols can also be advantageous in cross-coupling with acetylenes bearing electron-withdrawing substituents, such as propiolate esters, 115 which usually give unsatisfactory results under the standard conditions.
Cross-coupling to form carbon heteroatom bonds occurs by oxidative addition of an organic halide, generation of an aryl- or vinylpalladium amido, alkoxo, tholato, phosphido, silyl, stannyl, germyl, or boryl complex, and reductive elimination ( Scheme 2 ). The relative rates and thermodynamics of the individual steps and the precise structure of the intermediates depend on the substrate and catalyst. A full discussion of the mechanism for each type of substrate and each catalyst is beyond the scope of this review. However, a series of reviews and primary literature has begun to provide information on the overall catalytic process. 18,19,22,23,77,186
A series of interesting coordination compounds is involved in the cross-coupling chemistry described in this section. Because some of these complexes are included in other sections of the compendium, only selected compounds directly related to the catalytic chemistry will be discussed here. For example, Pd(PPh 3 ) 4 is a classic palladium(0) complex and a common catalyst. Its catalytic reactions involve well-known arylpalladium halide complexes. Yet, the arylpalladium thiolate, amide, and alkoxide complexes ligated by even common arylphosphines, such as PPh 3 , had not been prepared until recently. Moreover, isolated complexes containing ligands, such as the sterically hindered phosphines, that have been designed or selected to improve cross-coupling are less common. A few complexes containing these hindered ligands, such as Pd(P t Bu 3 ) 2 , are known, 187–189 but arylpalladium halide complexes of most of the most hindered ligands have been prepared only recently. 190
Several new palladium(0) complexes of t -butylphosphine ligands, such as those in Figure 4 , have been prepared. For example, the palladium(0) complexes of the parent ferrocenyl ligand 113 and of a biarylphosphine 191 that was present in Figure 3 have been characterized crystallographically. The palladium(0) complex of the pentaphenylferrocenyl ligand has been identified, but its structure has not been reported. 112
Figure 4 . Isolated palladium(0) complexes of sterically hindered alkylphosphines used in C–X coupling.
The oxidative addition of aryl halides to almost all palladium(0) complexes generates stable arylpalladium halides. The mechanism of this reaction has been studied in detail because of its importance to all types of cross-coupling. 55,77,186,192–202 However, the oxidative addition of aryl bromides and chlorides to Pd(P t Bu 3 ) 2 has been shown to be thermodynamically unfavorable in some cases. 203 This information does not preclude oxidative addition of aryl halides to Pd(P t Bu 3 ) 2 in the mechanism of the amination because the thermodynamics are likely to be only mildly unfavorable. In fact, reaction of an excess of PI with a combination of Pd(dba) 2 and P t Bu 3 or reaction of an excess of PhDr with Pd(0) complexes of P t Bu 2 (1-adamantyl), P t Bu 2 (1-adamantyl), and Ph 5 FcP t Bu 2 have all been shown to form metastable arylpalladium halide complexes. 190 The structures of the arylpalladium halide complexes bearing these ligands are unusual examples of arylpalladium halide complexes containing a single dative ligand. A weak agostic M H C interaction seems to contribute to the stability of these species.
During the cross-couplings to form C N, C O, C S, and C P bonds, the arylpalladium halide complexes are converted to arylpalladium amide, alkoxide, thiolate, and phosphide complexes. Examples of each type of complex have now been isolated, and the reductive elimination of the organic products has been studied. Although the reductive elimination to form carbon–hydrogen and carbon–carbon bonds is common, reductive elimination to form carbon–heteroatom bonds has been studied only recently. This reductive elimination chemistry has been reviewed. 23
Scheme 3 summarizes the reductive elimination chemistry of arylpalladium amides. Arylpalladium amido complexes containing PPh 3 as the dative ligand were stable enough to isolate, and they underwent reductive elimination of amine in high yield in the presence of PPh 3 . 36,204,205 The added PPh 3 serves to generate a stable palladium(0) product. Arylpalladium amide complexes of PMe 3 also undergo reductive elimination. In this case the reductive elimination occurs in competition with the formation of dimeric amido complexes. 206 Mechanistic studies of the reductive elimination of amine from the PPh 3 complexes 204,205 showed that reductive elimination occurred by two concurrent pathways, one involving reductive elimination from a three-coordinate intermediate formed by dissociation of phosphine, and one involving reductive elimination from a four-coordinate complex that is presumably the cis -isomer of the starting trans complex. The dimeric complexes underwent reductive elimination after cleavage to form a three-coordinate, monomeric, monophosphine amido complex.
Arylpalladium amido complexes ligated by chelating phosphines have also been prepared, and these complexes also underwent reductive elimination. 36,205 Studies of the complexes ligated by DPPF revealed important electronic effects on the rate of C N bond-forming reductive elimination. Complexes with more electron-rich amido groups underwent reductive elimination faster than those with less electron-rich amido groups, and complexes with more electron-rich palladium-bound aryl groups underwent reductive elimination slower than those with less electron-rich palladium-bound aryl groups. Reductive eliminations of N -aryl azoles, N -aryl benzophenone imines, and N -aryl hydrazones from palladium(II) phosphine complexes also occurred. Complexes of azolyl groups 93 underwent reductive elimination slowly because these groups are less electron-donating than are amido groups, while complexes of diphenylmethylenenimido ( N CPh 2 ) 93 and η 1 -hydrazonato ( NHN CPh 2 ) 99 ligands underwent reductive elimination at rates that were similar to those for reductive elimination of amines from arylpalladium anilido complexes because methyleneimido and hydrazonato ligands are about as electron-donating as arylamido groups.
Alkylnickel amido complexes ligated by bipyridine have been prepared that undergo reductive elimination of N -alkyl amines ( Equation (54) ). 207,208 Unlike the phosphine-ligated palladium arylamides, these complexes underwent reductive elimination only after oxidation to nickel(III). Thermally induced reductive elimination of alkylamines from phosphine-ligated nickel complexes appears to occur after consumption of phosphine by arylazides: 209
Arylpalladium and arylnickel thiolate complexes undergo reductive elimination of sulfides in high yields in many cases (Scheme 4) . 123,210–212 For example, [(bpy) 2 Ni(SR) 2 ] reacted with aryl halides to form aryl sulfide products, although an arylnickel thiolate complex was not observed directly as an intermediate. 123 Other work has focused on reductive elimination from isolated arylpalladium thiolates. 210–212 PPh 3 -ligated arylpalladium thiolates are dimeric and underwent reductive elimination in modest yields at 120 °C, 212 but isolated DPPE-ligated complexes are stable at room temperature in most cases and underwent reductive elimination of sulfide in high yields under mild conditions. 211 Reductive elimination occurred directly from this four-coordinate complex containing DPPE. Electronic factors again influenced reaction rates, although in a less pronounced manner than they influenced reductive elimination from the amido complexes. Arylpalladium thiolate complexes with more electron-donating thiolate groups underwent reductive elimination faster than those with less electron-donating groups, and complexes with more electron-rich palladium-bound aryl groups underwent reductive elimination more slowly than those with more electron-poor palladium-bound aryl groups. Various hydrocarbylpalladium thiolate complexes were prepared to determine the influence of hybridization of the palladium-bound carbon on the rate of reductive elimination. The rates for reductive elimination followed the trend, vinyl > aryl > alkynyl > alkyl. These relative rates have been explained by a combination of the coordinating ability of the π-system of the hydrocarbyl group and the amount of distortion required for this coordination. This explanation for the relative rates was supported by studies that revealed the effects of the steric properties of the thiolate and palladium-bound aryl group on reductive elimination.
The reductive elimination of ethers from arylpalladium alkoxide complexes has also been reported ( Schemes 5 and 6 ). 107,113,213,214 The effect of the electronic properties of ligands on the rate of reductive elimination of amines and sulfides suggests that the weaker electron-donating ability of the alkoxo ligand would make the reductive elimination of aryl ethers slow. Indeed, the first arylpalladium alkoxo complexes that underwent reductive elimination of ethers contained a strongly electron-withdrawing substituent on the palladium-bound aryl group ( Scheme 5 ). 107 Subsequent studies showed that reductive elimination of aryl ethers occurred in good yields from DPPF and BINAP-ligated palladium complexes generated in situ when the complexes contained electron-poor, palladium-bound aryl groups. 214 BINAP-ligated arylpalladium alkoxo complexes underwent reductive elimination faster than analogous DPPF complexes. Complexes with more sterically hindered alkoxo groups underwent elimination faster than those with less hindered alkoxo groups.
More recently, reductive elimination of aryl ethers has been reported from complexes that lack the activating substituent on the palladium-bound aryl group ( Equation (55) ). These complexes contain sterically hindered phosphine ligands, and these results demonstrate how steric effects of the dative ligand can overcome the electronic constraints of the reaction. 112,113 Reductive elimination of oxygen heterocycles upon oxidation of nickel oxametallacycles has also been reported, but yields of the organic product were lower than they were for oxidatively induced reductive eliminations of alkylamines from nickel(II) mentioned above: 215–217
Organometallic phosphido and phosphidoborane complexes undergo facile reductive elimination to form P C bonds ( Equations (56) and (57) ). One of the first examples of P C bond-forming reductive elimination was the formation of 1-diphenylphosphino-2-dimethylaminomethylferrocene from the ferrocenyl halide complex in Equation (56) and lithiumdiphenylphosphide. 218 A palladium phosphido complex was not detected during these studies, but a ferrocenylpalladium phosphide most likely forms the P C bond by reductive elimination. Recent studies have shown that reductive elimination to form the P C bond in phosphines occurs rapidly even from alkylpalladium or alkylplatinum phosphido complexes ( Equation (57) ). 219 Presumably, the strongly nucleophilicity and polarizability of the phosphido ligand causes these types of complexes to undergo fast reductive elimination. P C bond-forming reductive elimination from phosphido complexes of metals outside of the nickel triad, such as tungsten 220 and iridium, has also been observed: 221
Complexes of phosphidoboranes, instead of phosphides, have also been generated, and these complexes undergo rapid formation of triarylphosphineboranes ( Equation (58) ). 222 To generate a stable arylpalladium phosphido borane complex, a pentafluorophenylpalladium phosphidoborane complex was generated. This complex was stable enough at room temperature to be isolated and characterized crystallographically:
A reaction related to the cross coupling is the Heck reaction. The reaction involves the coupling of an aryl halide (or pseudo halide) with an alkene in the presence of a base. Owing to the latter, as in the cross-coupling reaction an equivalent of base is formed. In the base case, the Heck reaction will produce substituted styrenes. These products can also be made in the cross-coupling reaction discussed above. It is attractive that the Heck reaction does not involve Grignard-type reagents and thus it allows the presence of groups reactive toward Grignard reagents such as esters, acids, ketones, etc.
The reaction starts again with the oxidative addition of an aryl halide (Br or I) to palladium zero. The next step is the insertion of an alkene into the palladium carbon bond just formed. The third step is β-elimination giving the organic product and a palladium hydrido halide. The latter reductively eliminates HX that reacts with base to give a salt. Figure 60 shows the reaction scheme.
In modern Heck chemistry the halide is replaced by noncoordinating anions such as O 3 SCF 3 . The advantage is that the palladium center is now much less saturated and more positively charged (and hence more reactive) during the alkene coordination and insertion steps.
C.D. Varnado Jr. , C.W. Bielawski , in Polymer Science: A Comprehensive Reference , 2012
The use of transition metal-catalyzed cross-coupling chemistry in general and nickel in particular for the synthesis of condensation polymers was spearheaded in 1977 by Yamamoto and Yamamoto, 101 who applied the Kumada coupling reaction to the synthesis of poly(phenylene) and other poly(arylene)s ( Figure 16 ). These investigators were interested in poly( p -phenylene) because it is resistant to thermal degradation and therefore could be used as a material for applications that demand resistance to high temperature. Previous methods utilized in the preparation of poly(phenylene)s, including the Ullmann coupling and the Wurtz–Fittig reaction, were found to operate under harsh conditions (>200 °C, via radical pathways) to yield polymers that contained structural irregularity and branches. The Kumada coupling reaction was seen as a good alternative because it operates under relatively mild conditions. Indeed, such reactions are typically conducted by combining the various dihaloarenes with magnesium and the nickel complexes in THF followed by heating to 60 °C. A number of catalysts and ligands were screened to determine the optimal conditions; however, the polymers reported displayed poor solubilities and were of low molecular weight (the degree of polymerization values were found to range between 12 and 46 as determined by halogen content using elemental analysis and based on the assumption that both end groups were halogens). The inability to prepare high-molecular-weight polymers illustrated key challenges of this reaction for accessing polymeric materials, which would be later addressed by others.
Figure 16 . Selected poly( p -phenylene) (PPP) derivatives prepared via nickel homocoupling: poly(2,5-pyridinediyl) (top) and phenyl-substituted PPP (bottom).
Yamamoto et al . 28 first reported the Ni(0)-mediated homocoupling of aryl halides via a dehalogenation polycondensation process. Poly(2,5-pyridinediyl)s were prepared in good yields (54–95%) from 2,5-dibromopyridine, although the molec
Broken Asshole Porn
Girls With Long Nipples
Michelle Trachtenberg Nude Beautiful Ohio